6 Biodiversity, Evolution, and Extinction
Jason Kelsey
Biodiversity is a term that gets heavy usage among people in and out of the sciences. Simply put, it is related to the number of different species on Earth. You can get an anecdotal, visual idea of biodiversity the next time you go into a forest or other natural ecosystem: take note of the myriad of sizes, forms, adaptations, and survival strategies around you. From different types of trees, to grasses, shrubs, birds, insects, rodents, and all the rest, there is a great deal of variety readily visible in the biosphere. In this chapter, we will see that biodiversity varies with space and time due to many factors, including the processes of evolution and extinction.
Key concepts
After reading Chapter 6, you should understand the following:
- How biodiversity can be measured and defined
- How the theory of biological evolution is linked to biodiversity
- The ways organisms are related to each other and classified
- How the endosymbiotic theory can be used to explain the development of complex life forms
- The evidence for and examples of biological evolution
- The many factors that can increase and decrease biodiversity
- The phenomena that contribute to extinction of species
- The role of human activity in causing and mitigating extinctions
- The relevance of biodiversity
Chapter contents
6.1. The Definitions of Biodiversity
6.2. The Origins of Biodiversity
6.3. Biodiversity Varies with Space and Time
6.4. What are the Benefits of Biodiversity?
The Chapter Essence in Brief
6.1. THE DEFINITIONS OF BIODIVERSITY
Several varied, although linked, definitions of biodiversity have been developed and are used in different situations. Here we begin with a brief introduction to three widely used terms.
6.1.1. Species richness
This property refers to the total number of different species alive in a defined area. The biodiversity of Earth as a whole, as well as that of its individual ecosystems, can be assessed using this concept.
How many species are on Earth?
There is no universally accepted answer to this challenging question and no easy way to go about counting all the species on this planet. Estimates provided by ecologists during the past few decades have typically ranged from 3 million to 100 million, although a recent study that tried to include vast numbers of yet-to-be-discovered microorganisms put it close to 1 trillion[1]. Clearly, given the size of the error term in this measurement (Chapter 2), more research will be needed to come up with a reasonable answer. When only eukaryotes are counted, the number declines substantially to a range of approximately 8 to 10 million[2]. In any case, most readily agree that the number of species yet to be tallied exceeds the number we have cataloged already.
How many species are in a given ecosystem?
Species richness can be used to assess and compare different ecosystems. The total number of species in a tropical rainforest, for instance, is higher than that in a desert. It is also higher near an ocean coral reef than it is on the portion of a sandy beach that is only covered during high tide (Figure 6.1).
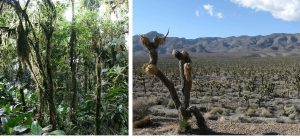
How broad or narrow are the niches in an ecosystem?
The relative size of the niches (Chapter 5) is a good proxy for species richness in any ecosystem.
Narrow. In this case, each species only carries out a small number of functions, lives in a very specific type of habitat, and is highly selective in choosing its nutrient sources. The presence of a large number of these specialists is a hallmark of high biodiversity.
Broad. Low-diversity environments are characterized by only a few organisms living in varied habitats and utilizing an assortment of different nutrient sources. In contrast to those in the preceding paragraph, low-diversity ecosystems are populated by generalists, species that are capable of a comparatively wide range of functions.
6.1.2. Species evenness
Species evenness is a measure of the relative number of different species present in an area, information that cannot be gleaned from an assessment of species richness. Put another way, evenness describes the balance among the species in an ecosystem. Keep in mind that richness and evenness do not depend on each other, that is, even if one is high, the other can be low. For example, a managed grassy field will likely have very low evenness because there are high numbers of only one or a few dominant plants, whereas an adjacent forested area with the same richness as the field could have high evenness, consisting of many individuals of multiple tree and shrub species.
6.1.3. Genetic diversity
A few words about genetics
Before we can consider how it can be used to quantify biodiversity, we need to take a brief look at the science of genetics.
Organisms are built from codes. Each living thing possesses a unique code in its cells. This code is stored in complex molecules known as deoxyribonucleic acid, or DNA. DNA molecules are long, yet microscopic, strands made up of shorter sections that are each called genes, and each gene controls the physical properties of a particular system. For example, different stretches of DNA contain the separate genes for human eye color, hair color, and skin color, as well as thousands of important traits related to growth, reproduction, and survival. The properties of all organisms are controlled by their DNA codes (also called their genomes), influencing which individuals are most likely to survive and thrive in their environments and which are not. Keep in mind that each time it needs to construct or repair a molecule or tissue an organism taps into the appropriate stretch of DNA. The term genetics broadly refers to all the information contained in DNA.
Appearances do not tell the entire story. Although all physical traits are a function of genetic sequences, not all data stored in DNA ends up translated into physical traits. Certainly the vast biodiversity on Earth results from differences in DNA, both small and large, but many organisms carry unused sequences, stretches of DNA that apparently are not used to construct any biological structure. Further complicating matters, some organisms having similar physical traits may have different genetic codes. In other words, it would be fair to say that genetic diversity exceeds physical diversity in the biosphere (particularly among bacteria, Chapter 3). Even members of a single species can differ substantially in their genetic sequences, despite appearances that might suggest otherwise. We will return to the distinction between physical form and genetic characteristics when we study biological evolution as well as how organisms are classified. This topic will also come up again in our discussion of genetically modified organisms (Chapter 9).
Genetic codes are passed from one generation to the next. Recall from Chapter 5 that reproduction is central to the continuation of living things. Although the details of how it is done vary from species to species, the general process is the same throughout the biosphere: mature individuals (we will informally call them parents) pass their DNA sequences to their offspring. For prokaryotes and other organisms that are asexual, a single cell will make a copy of its entire genome (termed DNA replication) before diving into two new cells. Under normal circumstances, each progeny cell is identical to its parent. In the case of sexual organisms such as humans, specialized reproductive cells are produced (called gametes, e.g., sperm and egg) which allow each separate parent to contribute half of the genetic code to an offspring. As a result, the new individual will have a complete genome that codes for a novel combination of traits; accordingly, it will resemble, not be identical to, its parents.
The meaning of genetic diversity
Now that we have gained the appropriate background, we are ready to consider how genetic sequences are used to characterize biodiversity of a population in an ecosystem. Genetic codes of individuals can be studied (it is no easy task to do this, but it is possible if the proper tools are available), and the degree of variation within a species determined (not the total diversity across multiple species). High genetic diversity would be indicated if a relatively wide variety of genetic sequences is detected. Many forces likely affect genetic diversity of a population. It is sometimes reduced in environments characterized by long-term stability because there is no pressure to adapt to new conditions on a regular basis—only a limited number of strategies are needed. Put another way, systems that have been subjected to a substantial amount of change and stress (from human and natural processes—Chapter 7) tend to display higher diversity within populations than those that are stable. As we will see below, ecologists tend to view high genetic diversity as a sign that a population is resilient and likely to successfully adapt to changing environmental conditions. These rules do not apply universally, and additional research is needed to better understand the factors influencing genetic diversity.
6.2. THE ORIGINS OF BIODIVERSITY
No matter how it may be measured and expressed, we can observe that Earth is populated by a wide range of organisms. What is less immediately obvious, though, is just how all that biodiversity came to be. This is not a new conundrum, for people have been struggling with it for millennia, although ideas and answers offered have changed with time and study.
6.2.1. The theory of biological evolution
This theory is the prevailing scientific explanation for Earth’s current biodiversity, offering an evidence-based mechanism by which the biosphere has developed. Its modern form started to take shape in the 1750s, but it has changed a great deal since that time. Like other theories (Chapter 2), it has been influenced by generations of researchers making objective observations, proposing hypotheses, taking measurements, and drawing conclusions. It has withstood over two centuries of scrutiny and continues to serve as a foundation for our understanding of life on this planet. Here we will explore the many principles and ideas comprising the theory of evolution.
Change with time
The term evolution refers generally to change during some time frame and can be applied to a wide range of entities, ideas, principles, relationships, and feelings. In short, anything that is dynamic and responsive to stimuli has the potential to evolve. Now, although most people understand the word to imply an improvement from a previous state, it is important to recognize that not all change is for the better, particularly when it comes to organisms. Yes, biological evolution is often characterized by changes that enhance a species’ ability to survive, but as we will see shortly, the story is more nuanced than that.
Processes affecting the evolution of organisms
The change in the inherited characteristics of a population is the essence of biological evolution. Put another way, modified genes and the physical traits controlled by them are passed between generations. Very importantly, evolution does not refer to or imply changes that occur during the lifetime of a single organism. Several processes interact and influence biological evolution.
Mutation. Recall that DNA contains the codes—the blueprint—that can be used to produce offspring. One of the assumptions underlying reproduction (both asexual and sexual) is that the complex genetic code stored in parents is faithfully copied before being passed to progeny cells. However, under many circumstances, replication is not perfect, and newly constructed DNA is different than the parental template from which it was built. In other words, the new DNA sequence contains errors or mutations.
Causes of mutations
DNA sequences can be changed via a number of mechanisms, the details of which are unnecessary in this discussion. Two broad types are very briefly described here.
External agents. As is known by many people, exposure to certain types of radiation, some toxic chemicals, and a small number of diseases can bring on mutations. We will see more about the effects of these types of agents on living systems in Chapter 15.
Endogenous errors. Mutations can also occur in the absence of any kind of external stressor. A phenomenon known as normal error can lead to changes in the DNA code during replication. The body can tap into several mechanisms to repair most errors before they cause trouble. Some mutations occur as a matter of course, though, and some of those progress into substantive outcomes for an organism.
Potential outcomes of mutations
Many outcomes are possible after the code has been altered.
Death of the offspring. If vital physical structures encoded by the mutated gene are affected, the organism will die.
Damage to the offspring. A wide variety of adverse consequences is possible. A non-lethal mutation often makes an organism less fit for survival and greatly reduces the likelihood it will reproduce.
No apparent changes. We know that for many organisms, in addition to genes employed to produce cells, tissues, and other structures, DNA contains information that is not translated into any physical traits (noted earlier). A mutation in one of those unused regions may lead to no obvious consequences.
Increased fitness. Although this outcome is rare, mutations can alter genes in ways that actually improve an organism’s ability to survive in its environment. Like those that damage an offspring, many specific mechanisms by which fitness is improved are possible. For example, as we saw in Chapter 5, DNA mutations can lead to antibiotic-resistant bacteria. An individual with an altered genome has the capacity to produce a new physical structure that enables it to survive exposure to penicillin or related compounds. It therefore has a great advantage over the cells around it that lack the mutation, and its offspring will dominate future populations (creating a public-health menace). Keep in mind that any kind of mutation increases genetic diversity of a population: more mutations lead to a greater variety of genes and possible physical traits in a group. Often, the total collection of genetic information stored in DNA of all the members of a population is referred to as a gene pool. In principle, as its gene pool gets larger and more diverse, a population becomes more and more adaptable, that is, able to survive under a wider and wider range of conditions. It follows, then, that a gene pool may contain many unused genes at any one time. Even a potentially beneficial mutation will not lead to an advantage until and unless environmental conditions change in such a way that the new traits provide a benefit to individuals possessing them. So, a bacterial cell that can withstand the toxic effects of, say, five different antibiotics, will only have increased success in an environment that contains those antibiotics. Many other examples of this phenomenon have been observed, as we will see shortly. We will also see that mutation is a powerful, but not the only, force contributing to evolution.
Recombination. Even in the absence of mutations, new genetic sequences can arise through this process. Put broadly, existing sections of DNA can be mixed together to create offspring with novel codes and traits.
Mechanism of recombination in two types of reproduction
Asexual. Individual microorganisms divide as described previously, providing a complete copy of their DNA to progeny cells (Figure 6.2a). Recall that interactions among different cells are not needed for reproduction of these organisms. However, cells still can exchange portions of their genomes under some conditions. Even though these interactions do not support reproduction, they do increase the diversity of the gene pool. Returning to the example we used above, microbiologists have noted how genes imparting antibiotic resistance can get passed to offspring as expected, but also among cells not engaged in reproduction. This transfer of DNA from one bacterium to another can occur both within and across species boundaries, further magnifying the scope of the problem for humans. Although seen rarely, some plants and animals can reproduce asexually as well.
Sexual. Each parent contributes to the code that ultimately makes up the genome of their progeny. Randomness in the selection of which portions of DNA actually move into sperm and egg, along with some other processes active during the formation of reproductive cells, lead to the construction of genetic sequences in the offspring that did not exist in either parent (Figure 6.2b).
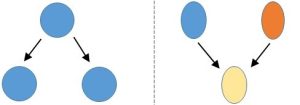
In general terms, recombination increases the size of the gene pool of a population. So, like mutation, it leads to gene sequences that could provide an advantage or disadvantage to affected individuals.
Natural selection. People often equate biological evolution with this process, but they are not the same thing. As we learned, evolution is the change in inherited traits in a population. Natural selection, on the other hand, is one of the processes influencing which traits are passed on from parents to offspring.
Natural selection is the way individuals of a species that are best adapted to their environments tend to succeed and reproduce at higher rates than less-well-adapted individuals. It can be informally viewed as a process that is able to sort out and rank individuals according to their fitness to survive. We have encountered this idea before. Recall from Chapter 1 that one of the fundamental concepts in environmental science is that organisms are tightly linked to and influenced by their environments. Pressures exerted by various conditions such as temperature, terrain, availability of food, competition and so forth shape organisms. Those possessing the most advantageous traits are most likely to pass their genes (and the resulting physical characteristics) into the next generation. Charles Darwin famously studied and proposed the basis for natural selection in the middle part of the 1800s, and his ideas, although revised and built upon by later scientists, remain central to the theory of evolution today.
Many factors influence natural selection
Since organisms are influenced by environmental stressors, it stands to reason that the traits providing advantages change as conditions change. In fact, what is beneficial to organisms at one point in time may not be sufficient to ensure survival at another. Several forces can change environmental conditions and therefore, the traits that are most helpful.
Hazards. Fires, earthquakes, volcanic eruptions, floods, and other events (Chapter 7) can quickly alter an environment, favoring individuals that are able to survive the immediate and long-term threats.
Slow changes. Environments are generally subject to gradual changes in temperature, moisture, and the like during long stretches of time: centuries, millennia, or more. Populations living under such conditions will need to adapt at a similarly slow pace.
Migration. Instead of enduring changes affecting their native habitat, a population may need to move to a completely different place. Drought, decrease in food supply, natural disaster, human activity, and competition are among the common stressors that can induce migration. Whatever the cause, pressure exerted by even subtle changes in environmental conditions in the new location will redefine which traits provide the biggest advantages to individuals that survived the journey.
Random chance. The phrase “survival of the fittest” is frequently used in reference to biological evolution and even has been known to make its way into social and business settings. It is founded on the principle that members of a population best adapted to their environments will necessarily be most successful, and weaker ones will fail. Surely natural selection does favor the best adapted individuals, but fitness alone is no guarantee of success. Under some circumstances, individuals least well adapted could be the ones passing their genes to the next generation. How could this anti-intuitive (and possibly upsetting) outcome occur? Random chance. Imagine, for example, a population of terrestrial animals living on an island. Because its favored food is most abundant in the surf zone, the water’s edge is the best place to live. Consequently, the dominant (i.e., most fit) individuals live closet to the ocean, and the weaker ones are forced to live inland on higher ground. Everything might unfold as we expect for a thousand years, with the near-shore organisms breeding most successfully and the inland organisms struggling to survive. However, one day a giant wave appears on the shore, instantly killing the members of the population gathered in the water. That random event gives an unexpected boost to the portion of the population safely living on top of the otherwise inhospitable mountain. These weaker individuals discover the shore is now open to them, so they come down to feed and breed. Fitness still plays a role in who succeeds and who fails, but this sudden change in the content of the gene pool deflects evolution away from the path we likely anticipate. We will explore inevitability (rather, lack thereof) further in the section below.
Important considerations and cautions
The theory of evolution is complicated and often misunderstood. Here we consider and clarify some important ideas, principles, and methods before we proceed further.
Many variables affect evolution. Biological evolution results from the interplay among the several processes described above. Specifically, mutation and recombination create new genetic sequences, potentially increasing the size of the gene pool, and natural selection and random chance dictate which genes dominate within a population.
Evolution involves heritable changes. As we saw earlier, biological evolution only affects characteristics that can be passed from one generation to another, not those that are acquired during an individual’s life. So, traits like eye color, hair color, height and so forth are heritable, but ability to play the piano or read a book or the loss of an ear due to an accident are not. A critical experiment conducted more than a hundred years ago helped to uncover this principle. In short, the tails were cut off rats after their births. Then, these altered rats were allowed to breed. Despite the condition of their parents, offspring were born with normal, complete tails. Even after many generations of similarly treated rats were tested (all unfortunate enough to have been relieved of their tails by researchers), newborns continued to possess tails. Scientists accept what seems like the most reasonable conclusion from these data: only the unaltered genes for tail formation were inherited, not the mutilation that occurred during the lifetime of parents.
Evolution produces new species. Interactions between modified genes and changing environmental conditions can ultimately produce a new species (a process known as speciation). Recall our understanding of the species concept (Chapter 5), to see an important characteristic of evolution: a group of better-adapted individuals that are unable to breed with members of the existing population can appear. This new species will likely become dominant because it does not mix its genes with less-fit individuals.
Biological evolution only applies to living things. Because it depends on genetics and reproduction, this form of evolution is unique to organisms.
Biological evolution is not predictable. Due to the role random chance plays in the process, as we saw above, it is impossible to predict the outcome of biological evolution. It might be explainable after the fact if sufficient evidence is available, but the nature of its output (what we might informally call its final product, assuming that evolution ever reached a conclusion) is by no means inevitable.
Relatedness is a function of common ancestry. You should realize that modern organisms are related to each other because of their shared ancestry, a relationship shown through a branching phylogenetic tree, not because one turned into the other (Figure 6.3.a and 6.3.b). For example, a widely held misconception is that humans evolved from monkeys. It turns out that all great apes (a group that includes us) are thought to have arisen from a single predecessor species that lived some 40 million years ago (Figures 6.3.c, 6.3.d., 6.3.e). Similarly, and perhaps surprisingly, modern hippopotamuses and whales also share a common ancestor. Again, hippos did not become whales, but two separate lines of descent branched from a single (now extinct) mammal about 54 million years ago. We will return to this topic later when we consider some of the evidence and data used to determine evolutionary relationships among organisms.
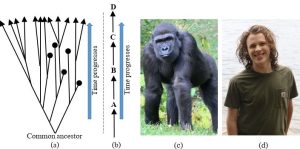
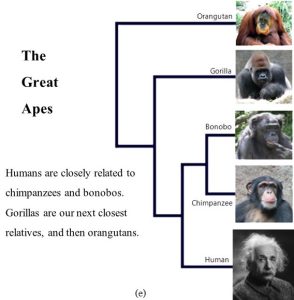
Scientists compare organisms to assess relatedness. Comparisons among organisms have long been used by evolutionary biologists to piece together the history of the biosphere. Similarities are assumed to be evidence of relatedness: close relatives ought to share more characteristics than distant ones (we will see it gets more complicated than that). Scientists have used two strategies to assess relatedness.
Physical traits
Historically, biologists compared the anatomy of different organisms to both determine how related they are to one another and to assign them to groups. One widely used organizational scheme divides the biosphere into five broad categories, or kingdoms: Animals, Plants, Fungi, Protists (which includes protozoa and some other eukaryotic microorganisms) and Monera (all bacteria). Those kingdoms are further divided into more specific groups. An underlying assumption is that organisms sharing many physical traits must have diverged from a common ancestor relatively recently. It is not always so simple, though. Firstly, similar physical traits do not guarantee close relatedness. Take, for example, two members of the animal kingdom, killer whales and great white sharks. In many ways, they look quite similar. But despite their shared characteristics, they arose from very different ancestors and should not be grouped together (we will see more about this example below, including images of the animals, in the section on convergent evolution). Secondly, it is not particularly straight forward to assess similarities between organisms lacking equivalent structures. How does one compare a barracuda to an octopus or a pine tree to a wolf, to cite just two confounding examples? What should be used as a point of reference? During the past century or so researchers have proposed and developed multiple approaches to improve comparisons, with varying degrees of success. Despite their efforts, the fact remains that an examination of physical traits only provides a limited amount of information about ancestry, relatedness, and evolution. As described in the next paragraph, modern biologists have been able to add a powerful tool to their studies.
Genetic sequences
Relatively new technology has enabled us to determine the genetic sequences of different organisms and, by so doing, make several discoveries. Notably, many of the genetic sequences within the gene pools of populations are conserved, that is, they remain largely unchanged for many generations. Even more importantly, it is now clear that a number of the same DNA sequences can be found throughout the biosphere, even in organisms that do not resemble each other physically. How is this information useful? As we learned earlier, genetic codes are passed from parents to offspring. In other words, the DNA in one organism is directly related to that in its ancestors. We also know, though, that sequences can change for various reasons during reproduction, leading to evolution as time passes. So, a scheme has been developed that uses comparisons of genetic codes to establish relatedness; new groups based on this scheme have emerged as well. The system assigns organisms to one of three broad domains (Figure 6.4): Eukarya (all eukaryotes), Bacteria (a group of prokaryotes that inhabit mostly familiar habitats), or Archaea (a group of prokaryotes that can be found in unusual, even extreme, habitats). Subdivisions within each domain have also been made, for example, within Eukarya are several kingdoms that each contain animals, plants, and many others.
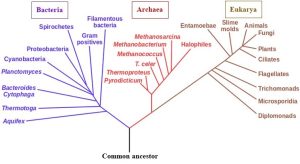
Determining relatedness through genetics provides many advantages, including the ability to study organisms with vastly different body types—the barracuda and octopus, again—and objectively compare and group them according to how well their DNA sequences match. It also bypasses potentially misleading information gleaned from studies of anatomy and focuses on a more reliable and relevant indicator of ancestry. As a result of our new understanding, older groupings based on physical traits have been revised to better reflect evolutionary relationships. Many instances of this reorganization could be cited, but we will only consider two of them here.
Monera get two domains. Because of their physiological similarities, traditional methods assigned all bacteria to a single kingdom, Monera. As we noted in Chapter 3, though, despite their somewhat limited variability in form, bacteria as a group possess an enormous amount of variety in life strategies and genetics. So, prokaryotes are now separated into two separate domains, Bacteria and Archaea.
People and fungi have a lot in common! It might be hard to imagine when you look at them side by side, but genetic analysis reveals how animals and fungi are closely related. Study Figure 6.4 again for more potential surprises.
Note that, despite lingering questions about some of its assumptions and methodologies, classification that uses gene sequencing is widely (if not universally) accepted among today’s scientists. Furthermore, most still consult both physical and genetic data in their studies of evolutionary relationships. See Box 6.1 for a bit more about the categorization of organisms.
Box 6.1. Where do we fit in?
Like all organisms, the species formally known as homo sapiens can be categorized using physical and genetic characteristics. Humans, along with chimpanzees, turtles, ants, trees, and fungi are all members of the same broad domain, Eukarya (Figure 6.4, above). Of that list, all but trees and fungi are part of the less general group, kingdom Animalia. The animals are further separated into subgroups (in increasing order of specificity, their phylum, class, order, family, genus and species). Chimpanzees and humans are similar enough to share the same family, but each is assigned to a different genus. Modern humans are the only living members of the genus Homo—all the rest are extinct relatives of ours such as Homo neanderthalis and Homo erectus. A final note is appropriate here. By convention, organisms are referred to by their genus and species names. In Chapter 5, for instance, we encountered the competitors Paramecium caudatum and Paramecium auerlia, so named because they are different species in the same genus.
Evolution of the biosphere requires a great deal of time. With few exceptions, including bacteria (Chapter 3) and insects (Chapter 9), evolution is very slow. Earth’s extreme age (Chapter 3), has allowed enough time for this gradual process to shape the biosphere
Biological evolution on Earth: some highlights
Earth is thought to be very old, forming about 4.6 billion years ago, and evidence of life dates back some 3.8 billion years (review Chapter 3, including Table 3.1). Clearly, it would be quite an undertaking to describe the entire history of the biosphere, so we will instead focus on just a few important highlights.
Biological molecules form (prior to 3.8 billion years ago). For living cells to develop, organic building blocks had to be available in the primordial oceans. Where did they come from? Evidence found in rocks and fossils suggests one commonly held answer. Briefly, Earth’s early atmosphere was very different than the one we observe today. There was much more methane, ammonia, and hydrogen cyanide, and no dioxygen, in the early days. These chemical differences matter because researchers have been able to observe the spontaneous formation of amino acids and other biologically relevant compounds from inorganic precursors under conditions thought to mimic those of ancient Earth. Other answers, including an extraterrestrial origin of biomolecules, continue to be studied and debated.
The first living cells arise (approximately 3.8 billion years ago).
The building blocks noted above started to cluster and organize
Recent experiments have demonstrated that basic cell components such as membranes and genetic material (Chapter 3 and the current chapter) are self replicating (i.e., can make copies of themselves), providing evidence that primitive units possessing many properties of living things, or proto-cells, could have given rise to the biosphere.
The common ancestor emerges
One of the underlying principles of evolutionary theory is that all organisms share the same origin. That is, modern scientists hold that the life-like entities noted in the previous paragraph became recognizable living cells and ultimately evolved into the diverse biosphere that has existed up through today. Put simply, every living thing on Earth can trace its history back to a common ancestor (again, review Figure 6.4).
The first cells were relatively simple
Evidence strongly suggests that the earliest organisms were single-celled, prokaryotic anaerobes that used the sun as their energy source (i.e., they were phototrophs).
Photosynthesis begins (approximately 2.5 billion years ago). Early organisms started to evolve and diversify into many species of prokaryotes. One of the most important changes occurred when certain organisms began to use the sun’s energy to fix their own carbon. Three crucial consequences followed.
Changes in atmospheric chemistry
Dioxygen (O2 gas), one of the products of photosynthesis, began to slowly accumulate in the atmosphere. By about 700 million years ago, the concentration of the gas had reached that of today, approximately 20% O2.
Changes in dominant organisms
We know that environmental conditions shape organisms. In this case, the release of gaseous oxygen by photosynthesis made the planet toxic to the anaerobic organisms that had dominated for so long. Anaerobes had to move into new environments that lacked oxygen, and the ability to use O2 became a substantial advantage everywhere else (review Box 1.1).
Formation of an ozone layer
Ozone is a gas made up of three oxygen atoms bonded together (instead of the two in the gas needed by aerobes). As we will see in much more detail in Chapter 14, its presence in the stratosphere (Chapter 4) protects organisms from much of the damaging solar radiation striking the planet. Without atmospheric ozone, organisms could not live in terrestrial environments. Indeed, life on Earth was restricted to aquatic environments before O3 appeared (water shields organisms from solar radiation). What does this have to do with photosynthesis? Well, ozone is formed through reactions involving dioxygen. So in the days before free O2 gas was present—prior to photosynthesis—there was no ozone layer and no terrestrial organisms. Again we see the mutual influences between Earth and life on Earth!
Eukaryotic organisms develop, and diversity of the biosphere increases (approximately 1.5 billion years ago). A quick look around suggests life on Earth did not stay restricted to single-celled prokaryotic forms. So, where did all these eukaryotes in your house come from? The short answer to the question is known as the endosymbiotic theory. There is a great deal of evidence to suggest that modern-day eukaryotes got their start when one ancient prokaryote ingested a relatively small prokaryote. Instead of being digested, this second cell was able to live symbiotically inside the larger one. The two organisms coexisted, and even provided benefits to each other. For example, the smaller one would have converted food ingested by the larger one into forms of energy both entities could use. They continued to reproduce independently, with one or more copies of the smaller cell being passed to subsequent generations of the larger one. Eventually, the descendants of the original cells became dependent on one another, setting up a permanent association that persists to this day. In other words, the organelles like mitochondria (Chapter 3) that are found inside of modern eukaryotes evolved from ancient, independent prokaryotes (Figure 6.5).
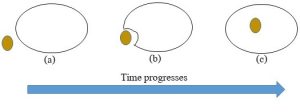
Although some people are uncomfortable with this theory, abundant evidence in support of it, including the six points listed below, has led to its wide acceptance among scientists.
1. Mitochondria are the same size as modern bacteria
Clearly this point alone is not enough to make the case, but it allows the idea to be plausible.
2. Mitochondria have their own DNA
The genetic information used to make copies of these organelles is separate from that of the main cell.
3. Mitochondrial and bacterial DNA are similar
As we saw briefly above, it is possible to determine the specific code of any strand of DNA. The results of such work are striking: sequences found in mitochondria have more in common with those in modern bacteria than they do with those in their own main cell.
4. Mitochondria reproduce independently of the main cell
These organelles copy their DNA and divide into offspring at their own pace, not necessarily at the same time the main cell divides.
5. Mitochondria and bacteria use the same type of machinery to build proteins
All organisms can construct proteins using specialized structures known as ribosomes. Although they have similar functions, prokaryotic and eukaryotic ribosomes differ from each other. Importantly, mitochondria possess prokaryotic ribosomes even though they are found inside eukaryotic cells.
6. Mitochondria can be sensitive to antibiotics
Recall that bacterial infections may be treated through the administration of chemical compounds that are selectively toxic to prokaryotes. Antibiotics work because they generally do not harm human hosts. However, mitochondria inside human cells are sometimes affected, again suggesting they are more closely related to prokaryotes than eukaryotes.
Multicellular organisms appear (approximately 700 million years ago). Up until this time, organisms were limited to unicellular or colonial lives (Chapter 3). With the addition of oxygen to the atmosphere, though, the stage was set for larger and more complex organisms to evolve. Through their use of O2, aerobes are able to extract far more energy from their food than are anaerobes. Since maintaining a large and complex network of cells (such as those in a plant or animal) is very energy intensive, multicellularity would have been far less probable if Earth had continued on as an exclusively anaerobic planet. The development of mitochondria and other organelles further facilitated the addition of multicellular organisms to the biosphere (for various reasons, including their ability to efficiently process energy sources). Extensive studies of fossils from different time periods have found a sudden (geologically speaking, of course) expansion of biological diversity about 570 million years ago, shortly after multicellular organisms first appeared (Table 3.1 in Chapter 3).
Organisms appear and disappear (ongoing). The history of life on Earth could be characterized as a series of trials and errors. Evolution can ultimately lead to the development of new species that persist for varying lengths of time. In some cases, a species is considered to be a dead end, a collection of traits and adaptations that did not provide meaningful long-term advantages. Some scientists use phrases like “failed experiment” to describe such organisms. However, time and again, even resilient, and seemingly permanent populations dwindle in size and become extinct; in the end, all that is left of them is fossil evidence (we will see more about the causes and consequences of extinction later in this chapter). Arguably, the story of dinosaurs is the most well-known example of this phenomenon. These animals appeared on Earth about 230 million years ago and dominated air, land, and seas for some 165 million years. Then, for reasons that are not entirely understood (likely a collision with a giant asteroid), they ceased to exist. Most believe an ability to adapt to rapid changes in environmental conditions was at least partially responsible, but the larger point is: species are temporary. As biological evolution shapes and expands biodiversity, extinction limits the number of different species present, as we will see in more detail shortly (see Box 6.2 for a bit of perspective on dinosaur history).
Box 6.2. These critters are OLD.
It’s time for another fun fact about dinosaurs and the vastness of history! The oldest dinosaurs (they arrived on the scene about 215 million years ago) are separated in time from the youngest dinosaurs (they went extinct about 65 million years ago) by MORE years than the youngest dinosaurs are separated from humans (appearing about 2 million years ago). You are encouraged to review Chapter 3 for more about geologic time.
Evidence in support of the theory of biological evolution
Much evidence has been collected to support this theory in the centuries it has been studied and scrutinized.
Increasing complexity in fossils. Multiple examinations of fossils have revealed something extremely important: relative simplicity of organisms increases with age of rocks studied. Study after study has found the remains of ancient organisms to get progressively more complex as one moves from oldest to youngest rocks. This trend broadly supports the timeline of evolution presented in the previous section (Figure 6.6).
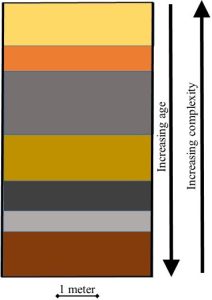
The universal genetic code. Despite the vast diversity in physical form found in the biosphere, all organisms employ the same basic strategy to store, process, and make use of genetic information. Among other commonalities, every strand of DNA is constructed of the same building blocks, whether it is in the cells of animals, plants, fungi, algae, or bacteria. Scientists view this to be a strong indication that all organisms originated from the same ancestor.
Similar developmental stages in separate organisms. The science of embryology, which studies the development of unborn (or unhatched) organisms, provides some interesting evidence in support of evolutionary theory and a common ancestor. In short, the embryos of related, but distinct, species are very similar, that is, they go through the same developmental stages. For example, a large number of mammal embryos possess gill slits and tails. Humans and other terrestrial organisms lose these structures before they are born, whereas aquatic organisms like fish retain them after they emerge (live birth or from eggs).
Homologous anatomical structures. Distinct organisms living in vastly different environments possess the same basic body plan and bone structure. For example, modern birds, lizards, rabbits, frogs, and humans, as well as some extinct organisms, all have the same three bones in their forelimbs, namely, the humerus, radius, ulna. Of course, the structures have been modified by evolution, but the data suggest these (and many other) animals descended from the same ancestor to have the same basic layout in their arms, wings, legs, and fins (Figure 6.7).
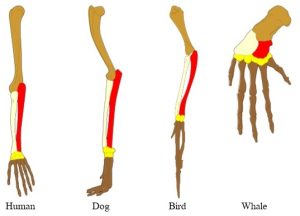
Existence of transitional fossils. One of the long-standing criticisms of evolutionary theory is that the fossil record lacks so-called transitional fossils, those that show organisms in the middle stages of evolution. However, recent research has uncovered multiple examples of fossils that possess traits of both earlier and later forms of organisms, indicating (but not proving, as we learned in Chapter 2!) a link, or transition, between them. One of the most dramatic and well-documented cases is that involving the evolution of whales (including dolphins)[3]. The history of these organisms has been confounding for centuries because of their unique combination of traits: they are confined to water, but, unlike fish, are mammals that have lungs for breathing. Such oddities led scientists to hypothesize that recent ancestors of these aquatic animals were land based, moving into the sea during their evolution. Examinations of fossils during the past century finally connected modern whales to a terrestrial animal that went extinct over 50 million years ago, but evidence of intermediate organisms, those that could document how these mammals transitioned to a permanent life in the ocean, had yet to be discovered. That all changed in the 1980s and 1990s, when paleontologists discovered fossilized remains of organisms that lived between 30 and 50 million years ago, the period during which whales were thought to have made their big move to water. These intermediate forms do indeed possess traits of both earlier and later forms, such as four leg-like limbs (fore and hind), tails, and ears adapted to hear underwater. As time went on, there is clear fossil evidence that bodies grew too big for the small legs that were shrinking but still present, and teeth became better and better for eating fish. The blowhole through which they breathed also moved from the front of the head to the top. Today’s whales have flippers for forelimbs (instead of legs), and only internal evidence of pelvic and leg bones (i.e., boney remnants of these former structures attached to their skeletons). Much has been written about whale evolution, and you are encouraged to consult the reference cited in this paragraph for more information.
Observations of evolution. Another common criticism of this theory is that nobody has ever observed evolution occur. Simply put, though, that widely promoted objection is incorrect: we have indeed witnessed natural selection and evolution unfold in real time.
Antibiotic resistance
Much about this phenomenon was described in Chapter 5 (review Box 5.6), and was also mentioned earlier in this chapter, so we will not repeat those details here. However, in this current context, you should realize that the development of bacteria able to survive exposure to poisonous substances is a direct result of rapid evolution. Through one of many possible mechanisms (some are explained above), the DNA in a subset of a bacterial population is changed—undergoes a mutation—such that the affected cells can now defend themselves against penicillin or another drug. Put another way, additional, potentially useful, information is added to the gene pool. If environmental conditions change and the bacteria are exposed to a relevant antibiotic, the cells with the new ability have an enormous advantage over all the others; due to natural selection, they reproduce and succeed whereas the sensitive cells perish. In a matter of hours or days, the population is dominated by resistant individuals. As we noted in Chapter 5, antibiotic resistance develops in natural environments, including human bodies. It can also be easily induced under laboratory conditions. If a population known to be sensitive to the effects of an antibiotic is grown in a container containing that substance. The few cells that survive are transferred to a second antibiotic-containing vessel and allowed to reproduce. Within days, a population of cells essentially identical to the original one (except now it is unharmed by the antibiotic) is thriving. In other words, such an experiment allows us to observe evolution directly.
Through a mechanism like the one active in the development of antibiotic resistance in bacteria, a population of insects can become resistant to the toxic effects of compounds that ordinarily kill them. Since these animals are quite a bit more complex than bacteria, the process typically requires a few years to occur (as opposed to a few days as seen in the prokaryotes). But evolution of these multicellular eukaryotes with relatively long and complicated DNA is still easily observable. We will return to this topic in Chapter 9 when we explore the environmental effects of agriculture and learn why, although it is a very interesting phenomenon and a nice illustration of natural selection at work, the development of pesticide resistance is not at all desirable or helpful to humans (it is great for the insects, though!).
Other experimental results
Many experiments have been designed to directly observe evolution. One such study conducted in the late 1970s showed how a population of guppies evolved in response to changing environmental conditions[4]. Since brightly colored male guppies attract both mates and predators in natural streams, the appearance of this fish will vary with specific conditions. In the presence of fewer predators, the colorful form dominates, but duller forms are prevalent in streams subject to heavy predation. Armed with this information, researchers set up artificial ponds to observe how the population would respond to various stressors. Notably, brightly colored males were most common when predation was absent. But when predators were suddenly added to those environments, the guppy population quickly changed to one dominated by dull-colored males. The experiment provides evidence that natural selection favors individuals with the greatest advantages. Here, those that are least likely to be eaten lived long enough to reproduce. Additionally, these results demonstrate how more information is carried around in the genome than is necessarily expressed through the physical appearance of a population (as we noted earlier in this chapter). In other words, these fish are able to quickly adapt because of what we might consider to be extra information in their gene pool.
Different types of evolution in biomes
In this final section on evolution we return to the concept of biomes we first encountered in Chapter 5 to consider three common and well-studied types of evolution.
Convergent evolution. Sometimes organisms in the same biome share many physical characteristics despite the fact that DNA analysis reveals they are only distant relatives. How could organisms with very different genes look similar? Because they live under the same conditions and stressors, distinct species often adopt common survival strategies. Put another way, they take on, or converge into, the same form (Figure 6.8).
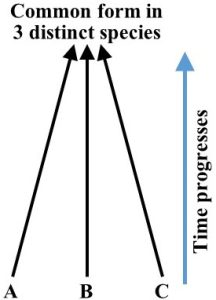
Examples of this phenomenon are not difficult to find. First, consider killer whales and great white sharks (mentioned above). Although they are both marine animals, they evolved from very different ancestors. Whales, as we know, can be traced back to a 50-million-year-old terrestrial mammal, whereas sharks and their predecessors are fish that have been evolving in the ocean for hundreds of millions of years. Genetic sequencing confirms these different lineages. Because they live in the same type of habitats (even overlapping in many places) and share many of the same food sources, though, they have a lot in common, notably, their size, shape, speed, strength, and sharp teeth. Certainly, there are differences as well, but their gross similarities are unmistakable (Figure 6.9).
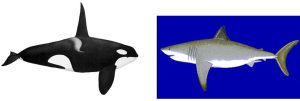
Second, birds and bats are also very distant relatives, yet they both evolved wings for flying to survive in their common habitat. Keep in mind that their wing structures are quite different from each other, though, suggesting they evolved from different ancestors. Multiple pieces of evidence suggest that birds are more closely related to crocodiles than bats, and bats, in turn, are far more like mice than birds. Finally, convergent evolution can even be seen across millions of years. The extinct dinosaur triceratops and the modern rhinoceros have very little in common genetically: they arose from distinct ancestors (i.e., rhinos did not descend from dinosaurs). However, to survive the same types of environments, food sources, and other stressors, they took on rather similar physical forms (Figure 6.10).
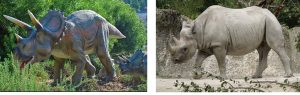
Divergent evolution. In this second case, a single population splits into a few distinct, yet closely related, species living in separate places and biomes. That is, the reason different species resemble each other is their common genetic origin, not because they adapted to the same stressors in the same place (Figure 6.11—compare it to that for convergent evolution, Figure 6.8).
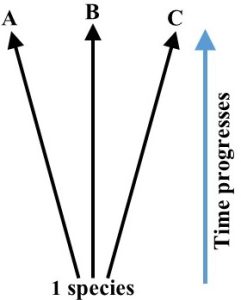
Migration, volcanic or earthquake activity, or even the breakup of Pangaea and movement of continental masses (Chapter 3), could cause groups within a population to live and evolve under different environmental conditions. Results of divergent evolution can be seen throughout the biosphere. For example, the kit fox, arctic fox, and the red fox are three closely related species that emerged from a single ancestor[5]. The kit evolved in desert environments, developing a sandy color and relatively large ears to help dissipate heat, the arctic developed a white coat in snowy areas, and the red is adapted to life in the forest, where its color allows it to stay hidden among trees (Figure 6.12).
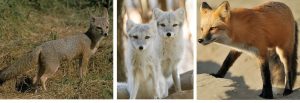
The divergence of monkeys and great apes from a common ancestor some 25 million years ago is another good example of this phenomenon. These many species share much of the same DNA sequences (well over 90% in most cases) yet evolved distinct sizes, lifestyles, and other traits in response to differences in the stressors acting on them.
Adaptive radiation. This process is like divergent evolution in that many closely related species arise from a common ancestor. A wheel is a better model for adaptive radiation, though, because competition for resources can cause individuals of a single population to evolve specialized survival strategies and an appreciable increase in the number of related species in a particular area (Figure 6.13., left panel). Changes in the gene pool and natural selection lead to distinct new species with the capacity to exploit multiple, narrowly defined niches (i.e., each has a particular set of features that allows it to play only a small, distinctive role). Every population uses a slightly different strategy to survive, so the restrictions of the competitive exclusion principle are eliminated (Chapter 5). In short, similar organisms can exist side by side. The most famous example of this phenomenon is, arguably, that reported by Charles Darwin in 1859. While studying in The Galapagos Islands, he saw a large amount of biodiversity. Notably, the many different species of finches (a type of small bird) living there possessed similar, but not identical, physical features. After much research he proposed that such variety was the result of evolution from a common ancestor. Because of intense intraspecific competition (Chapter 5), individuals able to find food in novel ways have an advantage over others. He described many small niches occupied by species with different forms. To name just some of the fourteen recognized, beaks specialized for hunting of surface insects are different than those used by species that tear open plants to find food, whereas nut eaters have a thicker, stronger beak to break open hard shells. Again, both visual inspection and gene sequencing reveal that these birds are very close relatives that evolved from a single species (Figure 6.13., right panel).
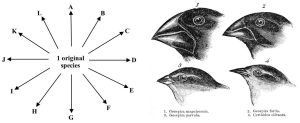
6.3. BIODIVERSITY VARIES WITH SPACE AND TIME
Some areas seem to be obviously teeming with many different forms of life whereas others are dominated by a very small number of species. In addition, careful examination of fossil evidence reveals that species richness has gone up and down during the planet’s history. Here we briefly consider some of the explanations for these observed variations in biodiversity.
6.3.1. Factors increasing biodiversity
Processes favoring evolution and therefore speciation tend to increase the number of different species present in an ecosystem. Keep in mind that these are merely generalizations, and multiple factors can interact to bring about unexpected results.
Physically diverse habitats
Anything that increases the number of different types of habitats within an ecosystem will stimulate populations to develop unique survival and life strategies. So, a flat and relatively uniform plain or ocean floor will be likely less diverse than a rocky, hilly area or coral reef zone, respectively.
Moderate disturbance
This factor is tightly linked to the previous one because a relatively minor event such as a small fire or flood can increase habitat diversity and favor new adaptations and species.
Middle successional stages
As we saw in Chapter 5, ecological succession changes the dominant species in an area. Generally, early- and late-stage communities tend to be less biodiverse than the middle, transitional ones.
Long-term environmental stability
This factor might not seem to fit our pattern at first, but environments that do not change for millennia or more can encourage the development of increasing numbers of species as they age. Ecologists generally agree that such stability allows for experimentation in the biosphere and a narrowing of niches (as described above). Viewed another way, all available niches can be exploited if evolution is given sufficient time. Diverse tropical rainforests provide a good example of the effect of stability on biodiversity. Since sunlight and other nutrients are abundant near the equator, organisms are productive all year long. Resultant near-constant interactions push competitors to develop new strategies to survive. The adaptive radiation seen in Darwin’s finches demonstrates a nice specific case of how stability can lead to specialization.
High diversity in low trophic levels
Through one or more of the mechanisms noted on this list, biodiversity among organisms at the first trophic level can increase. Higher-trophic levels will then likely become more diverse and specialized in response.
6.3.2. Factors decreasing biodiversity
Anything that increases rates of disappearance of existing species relative to those of the evolution of new species will decrease biodiversity.
Extreme environmental conditions
Only a few species are likely to have the capacity to adapt to and thrive in unusually challenging habitats. For example, very low or high average temperatures, a wide range of possible temperatures from lowest to highest, excessive or scarce nutrients or water, or extremes in other properties including those related to chemistry, light, other forms of radiation, or atmospheric pressure tend to create conditions that are at the limits of tolerance for organisms. As a result, biodiversity in such places is typically quite low, with ecosystem functions carried out by the few generalists that can survive under such stress. For example, deserts, the tops of tall mountains, the floors of very deep oceans, and polar regions are simply too harsh for many species to develop the traits necessary for success.
Extreme disturbances
Events like volcanic eruptions, severe flooding, and human construction can disrupt natural systems and vastly reduce biodiversity. If succession is eventually allowed to proceed, diversity could increase. However, when a system undergoes long-term and persistent change, say, a human-induced transformation to a farm, park, golf course, or neighborhood, biodiversity is likely to remain limited.
Invasive species
As we saw in Chapter 5, certain exotic organisms known as invasive species are better suited for survival than are species endemic to an area. Since they grow more quickly and aggressively, these outsiders outcompete the natives and take over an ecosystem in a short period of time. Both species richness and evenness may decline precipitously after invasives appear.
Extinction
A species that is no longer present on Earth is said to be extinct. Now, things get more complicated from here because the circumstances leading to extinction vary, and many forces can contribute to the loss of a species. Adding to the confusion, it can be difficult to know if an organism is extinct or simply rare—many species have seemingly disappeared from forests, prairies, lakes, oceans, and other ecosystems only to be seen alive and well (albeit scarce) after escaping observation for decades. We explore some of the causes and consequences of extinction below.
Small populations are imperiled. Any phenomenon that reduces numbers can indirectly lead a seemingly robust and successful species to extinction because small populations are inherently susceptible to total elimination. Put another way, it is unlikely that a single cause will directly kill off every member of a population, rather, low abundance creates its own risk. Two important reasons small populations go extinct are briefly described here.
Population risk
Numbers may be, in principle, high enough to maintain a healthy population indefinitely. In practice, however, the rate of reproduction is too low to keep up with death rate because individuals simply do not interact regularly enough to produce offspring. It comes down to density: the chances of two fertile individuals finding each other declines as members of a species are spread more and more sparsely within a large habitat (see Figure 6.14). If you picture, say, a handful of whales trying to locate mates in the world’s enormous oceans, you can appreciate the way population risk hastens extinction.
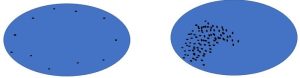
Genetic risk
A population may get so small that closely related individuals have little choice but to breed with each other. This phenomenon, known as inbreeding, weakens a species because it tends to raise the number of congenital defects passed to young. Why is inbreeding risky? As we learned earlier, DNA mutations do not always lead to adverse physical traits. Realize, though, that for various reasons such errors can stay masked and have no obvious effect on an organism that carries them. A union of two organisms having similar genetic codes (complete with the same unexpressed mutations) is far more likely to yield poorly adapted offspring than is one between unrelated parents (i.e., those with dissimilar DNA). Subsequent generations are increasingly unfit to survive, as mutations become ever more prevalent, numbers drop further, and extinction ultimately occurs. High genetic diversity, therefore, is more beneficial to the long-term survival of a species. Consult Box 6.3 for an example of the effects of inbreeding among humans. Genetic risk can be particularly important for isolated populations such as those on small islands or are otherwise segregated (see Figure 6.15 for one example).
Box 6.3. Risky royal reproduction
To maintain a sort of genetic purity (or at least the perception of one), some royal families have deliberately reproduced with close relatives. Unfortunately, this once widespread practice led to unintentional consequences: many offspring of those unions were born with debilitating congenital diseases. For example, the Spanish Hapsburgs (16th – 17th Century) were a dynasty that died out due to inbreeding. As a result of a few generations of marriages among people with similar genes, they got weaker and weaker until Charles II, mentally and physically disabled, died without leaving an heir in 1707[6]. Some of the descendants of Queen Victoria of England (19th Century) experienced a similar fate. The genetic disorder hemophilia (a blood-clotting disease that was largely untreatable a century ago) was prevalent in her family tree. Among those affected was the son of Alexandra (she was a granddaughter of Victoria) and Tsar Nicolas II of Russia (he was one of Alexandra’s cousins). You have likely heard of Nicolas, as he was famously murdered along with his hemophilic son and the rest of his family in 1918 by Bolsheviks—but you will have to consult a very different kind of book for more about them.
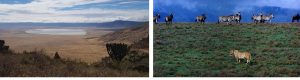
Forces reducing population size. Although humans certainly play an outsize role in driving extinction of organisms these days—we will explore many of the ways they do that below and in upcoming chapters—it is worth noting that natural processes have been responsible for nearly all extinctions during Earth’s long history. The demise of the dinosaurs some 66 million years ago, for example, took place at least 64 million years before beings resembling homo sapiens even appeared (and some 66 million years before modern humans began to exert their influence). This famous extinction is just one of the hundreds of millions that have occurred since life first arose. It would be fair (and possibly humbling) to say that every organism is subject to extinction sooner or later: data indicate that on the order of 99% of all species that have ever lived are now extinct! But it is a complex and nuanced phenomenon, as species persist for different lengths of time, and the rate of loss from the biosphere has ebbed and flowed substantially. Although extinction appears to occur continually, scientists have used fossil evidence to identify five distinct and relatively short-lived periods in the past 600 million years, known as mass extinction events, during which an unusually large fraction of species, in the range of 75% or more, living at the time went extinct. The most recent of these is thought to have been the one that ended the 165-million-year reign of the dinosaurs. As we will explore below, a devastating mass extinction event may well be under way today.
Natural environmental change
We have seen multiple times (for example, in Chapters 1, 3, 5, and earlier here) that organisms best adapted to their environments are most likely to survive and reproduce. It should come as no surprise, then, that changes in conditions due to natural processes can jeopardize the ongoing success of previously dominant individuals. Generally, the higher the rate of change the more difficult it is for organisms to develop necessary adaptations for ongoing survival.
Sudden. Some phenomena are just too sudden and powerful for organisms to survive. Volcanic eruptions, earthquakes, tsunamis, infectious diseases, and asteroid impacts are all examples of agents that can devastate populations, communities, or entire ecosystems. Whether an event leads to extinctions depends on the scope of the damage it causes as well as the uniqueness of the affected habitat. Imagine an organism so specialized that it is only found in one small area on Earth. In such a case, a single volcanic eruption, with its lava, poisonous gases, and ash (Chapter 7), could so dramatically reduce numbers that extinction occurs. The global-level effects of a collision with a large asteroid, however, could imperil even abundant, widely distributed species.
Gradual. Slow alterations to an environment may cause a dominant organism to be replaced. Climate change due to natural forces, increased predation or competition (Chapter 5), disease, or other stressors could all drive species that cannot adapt to the brink of extinction.
Anthropogenic activities
Before exploring this topic, a note about the organization of this book is appropriate. You might recall from your reading of Chapter 1 that Part II (Chapters 4 – 6) was advertised as an examination of natural phenomena with little attention devoted to human sources of stress. Here we temporarily deviate from that scheme because a discussion of extinction would be incomplete without some explanation of the roles played by anthropogenic activities.
The numerous and varied ways humans contribute to extinction can reasonably be placed into one of two categories. The first of these, direct, involves activities that are intended to kill or capture organisms. Indirect causes, those in the second category, do not set out to harm organisms, instead, they initiate changes that ultimately endanger survival of a species. The motivation for an activity, in other words, may be used to distinguish between these categories of extinction. We will keep things general for now but explore many specific human activities and their consequences in Chapters 8 – 14.
Direct causes of extinction. Hunting and harvesting are two familiar activities that seek to remove organisms from their habitats. The issue is nuanced, of course, because the killing of organisms that are extremely abundant does not necessarily lead to population risk. For instance, white-tailed deer reproduce so quickly that hunting of these animals does not even maintain a stable population in many areas—their birth rate is just too high for hunting to keep up. Large numbers do not ensure resilience, though, as in the famous case of the passenger pigeon. As recently as the Nineteenth Century, many billions of these birds lived in North America. High-density flocks containing millions of birds—the skies were said to darken when they flew overhead—were such tempting and easy targets that human hunters drove them to extinction a little more than a century ago[7]. The Bengal tiger of India provides one modern-day example of an animal in danger of going extinct. Since its numbers are already quite low, laws have been passed to protect and conserve the cat. Ongoing hunting (or, more appropriately, poaching), along with other stressors described in the following sections, continue to affect this and similarly threatened organisms.
Indirect causes of extinction.
- Habitat destruction. By modifying or eliminating natural ecosystems to make space for housing, recreation, industry, commerce, and farms, humans can introduce sufficient stress to drive species to extinction. The problem is more complicated than it may appear because the effects of development depend on factors such as whether an organism is a generalist or specialist and how unique is the affected habitat. An organism with a broad niche (Chapters 5 and above)—say, rats (clearly, they can live about anywhere humans live)—will be far less vulnerable to alterations than would one with a narrow niche—say, giant pandas (an animal having very quirky habitat needs and reproductive habits that, even absent human activities, hinder their long-term survival). For our purposes, “destruction” can refer to dramatic replacements of natural systems by human ones; think clearing a dense forest and replacing it with a shopping mall or agricultural field.
- Habitat fragmentation. Seemingly subtle changes and modest losses of natural habitat could profoundly affect vulnerable organisms. Although a construction project might convert just 10% of land into a highway or pipeline (Chapter 10), the presence of this unnatural barrier could interrupt migrations, reduce genetic diversity in a small region, disrupt seed dispersal and pollination, or otherwise impede behaviors necessary for a species’ survival. For example, a single forest split into disconnected sections by roads, railroad tracks, parking lots, recreational areas, or other anthropogenic features could lead to the extinction of an organism that requires large areas of continuous space to be successful (Figure 6.16). Dammed rivers and divided wetlands can similarly harm an organism.
Figure 6.16. Human development can lead to habitat fragmentation and reduce the success of a species. The addition of roads and houses creates more and more barriers with time (top diagram, in map view). These highways in Indiana have disrupted the free movement of animals (bottom photo, map view). Kelsey, CC BY-NC-SA (top); USGS, Public Domain (bottom). - Habitat degradation. Humans may not appreciably alter the appearance or continuity of a habitat, but they still can decrease its suitability for organisms that depend on it for survival. Water pollutants (Chapter 11), direct dumping of toxins (Chapter 13), or release of air pollutants (Chapter 14) can stress organisms living in a region. Somewhat related to habitat degradation: actions that have a direct impact on one trophic level could indirectly harm other organisms in a community. For example, by killing members of a prey species, humans might decrease the amount of necessary nutrients available to a high-level predator.
Consequences of extinction. The disappearance of an organism from Earth can trigger consequences ranging from subtle to severe.
Biodiversity decreases
As stated above, each extinction reduces the biodiversity in an ecosystem (and, for that matter, in Earth’s biosphere). The relevance of such changes is addressed later in this chapter.
Ecosystem structure changes
To understand this effect of extinction, recall that we defined structure as the identity of the organisms present in an ecosystem (Chapter 5). Clearly, when a population disappears, the inventory of species is modified. Effects of such changes can vary, as we will see in the next item.
Ecosystem function may change
Extinction can alter this second ecosystem characteristic, although its effects are not always dramatic or obvious. On the one hand, a loss (i.e., altered structure), could bring on catastrophic changes if members of the affected population carried out a critical in an irreplaceable way. Such an organism is often called a keystone species, one that has a disproportionate effect on the survival of an ecosystem (relative to its abundance). Consider, for instance, the potential effects of extinction of a high-level predator: among other things, the growth of prey species could accelerate and lead to habitat degradation. The sea otter in waters off the Pacific northwest of the U.S. is a keystone species because it preys upon sea urchins that would otherwise destroy kelp forests[8]. The white nose bat is also a keystone species because it plays such crucial roles as both predator and prey in its ecosystems (largely northeastern United States). Recent declines in bat numbers are of great concern because extinction could devastate the ecosystems it inhabits[9]. Alligators in swamps in southeastern U.S.[10], gorillas in Africa[11], and oak and hickory trees in the central hardwood forest (east-central U.S., including Tennessee)[12], are all examples of keystone species. On the other hand, some organisms are less crucial for the survival of entire ecosystem because their function, although necessary and important, can be carried out by another in largely the same way. In principle, removal of such an organism could enable a second species to grow and expand to ensure the overall survival of an entire ecosystem. Commercial fishing (Chapter 12) is one activity with the potential to exert so much pressure on one species that others having less economic and nutritional value for humans could take over. In such a case the whole ecosystem would suffer minimal changes, yet people would be harmed due to the loss of a valued commodity. We will see more about the effects of extinction on humans below.
Human civilization can be affected
Loss of important ecosystem services. Humans depend on many processes active within ecosystems, including the release of dioxygen by primary producers, nutrient and water recycling, decomposition, and trophic interactions. Extinctions can disrupt these services by changing ecosystem structure and function.
Loss of economically valuable products. Since extinction is an irreversible process, it could lead to the permanent disappearance of organisms having medical, industrial, or commercial uses. The Pacific yew tree is one example of the way extinction could bring on an undesirable outcome from an anthropogenic perspective. In the 1980s, scientists discovered that paclitaxel (known as Taxol commercially), a chemical substance derived from the tree’s bark, could treat human cancers. Subsequent high demand for this drug, as well as other stressors from human development, have reduced the size of the yew’s population. Although numbers have not yet dwindled to critically low levels, the potential adverse outcome of its extinction is likely obvious: loss of this tree would mean no more Taxol[13]. Unsustainable harvesting of fish (known as overfishing, a phenomenon we will revisit in Chapter 12) could similarly lead to the loss of a source of both income and food. Reductions in the availability of valuable organisms could also lead to conflicts among nations and peoples. Finally, high biodiversity attracts tourists (and their money) to areas having unique ecosystems. For nations that are heavily reliant on income from visitors seeking exotic wildlife, extinctions might equate to job losses, increased poverty, and crime.
Subjective considerations and losses. Obviously beyond the scope of science, these are important to many people. Put simply, human-driven extinction is seen by some as morally unacceptable. Although not objectively measurable, concerns about the rights of non-human organisms to survive as well as aesthetic and cultural desires to maintain Earth’s current biodiversity inspire interest in preserving endangered organisms.
Steps can be taken to slow extinction. Many laws enacted during the past several decades are intended to counteract or reverse human contributions to extinction. In the United States, for example, President Theodore Roosevelt helped establish the first national wildlife refuge to protect threatened water birds in 1903. In the years that followed, numerous other related actions were taken by the federal government, including the passage of the Endangered Species Act (ESA) in 1973 (with amendments in subsequent years). In short, this law uses scientific data to identify at-risk species and empowers the Fish and Wildlife Service (FWS) to formally designate them as endangered or threatened. These two categories reflect a matter of degree: organisms in the former are in imminent danger of complete disappearance and those in the latter have dwindled enough that they could become endangered. Listed species are protected by restrictions on hunting, harvesting, or any actions that directly harm them (referred to as take in the language of the law), as well as human activities that would damage their habitat or otherwise indirectly threaten them. Many plants and animals have been added to the list of endangered and threatened species since the ESA was first enacted, including the American alligator, American peregrine falcon, and the San Clemente Island Indian paintbrush[14]. The alligator and falcon have since recovered sufficiently to be delisted, but the paintbrush shrub is still considered threatened by the FWS (we will see other examples shortly). State and local agencies in the U.S., as well as international organizations, also pass laws to protect imperiled organisms.
Protecting species poses multiple challenges, especially when such efforts are perceived to conflict with human quality of life. Should we restrict logging in a unique ecosystem because it endangers the survival of a (seemingly) obscure insect or bird? The people who earn their living by cutting down trees often answer with an unequivocal “no” (more in Chapter 1)! Similar questions can be asked about many other potentially threatening endeavors such as development of new housing, expansion of agricultural land (Chapter 9), exploitation of fossil fuel resources (Chapter 10), and commercial fishing (Chapter 12).
Here we take a generalized look at the protection and recovery of endangered and other at-risk species. We will see some important example organisms and the specific measures taken to protect them in the next section.
Identifying a change in population status
Scientists working for various governmental agencies conduct surveys of organisms and ecosystems to monitor trends in population sizes and stability. If a species appears to be declining, a decision is made about changing the way it is regulated, that is, whether it should be added to a formal list of endangered or threatened species (compiled by U.S. state and federal agencies and at the international level). A species on one of those lists gains special protections against measures that could further imperil it (including hunting, commerce of products from it, and land use decisions that adversely affect its habitat).
Determining the cause of decline of a species
We need to determine what is limiting success—lack of suitable habitat, new competitors, moisture stress, pollution, excessive hunting, to name just some of the possible stressors—before we can do anything to reverse an observed decline.
Devising a recovery plan
Identifying the underlying problem is often a challenge in its own right, but taking the necessary next steps can be even more difficult. Often, unpopular steps are required, including the imposition of restrictions on harvesting and hunting, the establishment of protected areas, and the tightening of laws regarding new construction. In any case, the development of a plan to help an endangered species requires answers to several questions, including those described here.
What is our objective? This seemingly simple question can be, in practice, very difficult to answer. Of course we want the size of a target population to increase to healthier levels, but determining what that number might be presents some challenges. How big is big enough? Because of divergent points of view and agendas, different people will come to different conclusions from the same data about a target organism. In many cases, it is impractical for a population to rebound to some historical size because humans now live in its former range. Do we really want gray wolves to return to the geographic locations that are currently villages and cities? Assuming most people would opt out of sharing space with dangerous predators, we need to settle on a less-ambitious goal like small but sustainable populations living in remote areas (more about wolves follows shortly).
What steps should we take? The answer to this question is highly dependent on the species of interest, its habitat, and the causes of its decline. If it is a commercially important fish, restrictions on harvests are clearly in order, whereas protection of a marine mammal that is not deliberately caught but gets killed inadvertently by gear designed to capture fish would likely involve changes to fishing practices (Chapter 12). Elephants, rhinoceroses, lions, and bison benefit from restrictions on hunting and poaching as well as habitat protection. Banning the trade of things like ivory is also intended to enhance survival of protected species. Endangered insects, worms, plants, and other types or organisms obviously require varying species-specific approaches. Wildlife refuges and zoos can be parts of recovery plans, although their utility and appropriateness are not universally recognized (see Box 6.4 for more).
Box 6.4. What about captive animals?
Animals (and, to a lesser extent, other organisms) are often taken from the wild and kept in zoos and aquaria. A related, but clearly different approach, involves large areas commonly called wildlife refuges in which organisms are protected and live under conditions that are very similar to their natural habitats.
1. Zoos. Animals are put on display in enclosures that may bear some or little resemblance to their natural habitats. Arguments are made in favor of and against their usefulness. On the plus side, their captive breeding programs can be important components of recovery plans for listed organisms. For example, the U.S. Department of Fish and Wildlife (FWS) has a relationship with the American Association of Zoos and Aquariums that, among other things, takes advantage of the capacity of zoos to increase reproduction of endangered species[15]. Furthermore, many hold that zoos educate and inspire people to contribute to conservation efforts. Detractors contend that zoos are unrealistic displays of inhumanely imprisoned animals and teach little about the value of conservation (e.g., African zebras in a snowy pen in Pennsylvania, U.S.A.???). Some see zoos as an inappropriate allocation of resources that could otherwise be spent on more meaningful conservation efforts of organisms in their natural environments.
2. Wildlife refuges. These areas are intended to keep organisms in natural conditions. Put another way, rather than removing an animal from an African savannah and putting it into a glass-enclosed cage in Washington, D.C., efforts are made to protect organisms in their native habitats and enhance their ability to reproduce and thrive in place. Refuges have been set up in many countries. The U.S. FWS oversees several hundred such places throughout the United States and its territories. Since they address site-specific needs, the details of their operations vary considerably. In general terms, they are maintained to conserve natural species and habitats, including those that are endangered or threatened. You may be surprised to read that refuges often are not closed to the public. Hiking, swimming, picnicking, hunting, fishing, and harvesting tend to be allowed, with regulations and controls, in these protected spaces. Other examples of refuges are seen in multiple African nations stiving to strike a balance among hunting, farming, tourism, and conservation. The U.S. FWS is one of the partners that support the work carried out by the Central African Regional Program for the Environment (CARPE) to maintain and protect animals, plants, and ecosystems that are threatened by hunting for both trophies (e.g., elephant ivory and exotic pets) and food (e.g., the flesh of great apes and other large animals or bushmeat), grazing by cattle, mining, logging, and other anthropogenic activities[16]. Their focus on in-place conservation and the needs of native peoples distinguish refuges from zoos, although the potential for abuse and corruption, among other weaknesses, make them an imperfect solution.
Important final thoughts. Before we move on, two additional comments are in order. First, our approach to protection has changed during the past several decades. Historically, efforts focused on a single species but, given our understanding of how organisms interact with and rely upon their surroundings, modern scientists often target whole ecosystems (more below). Rather than exclusively tracking and helping, say, a particular endangered turtle, we strive to understand the living and non-living factors upon which that turtle relies and conserve them. Many factors must be studied, such as whether (and how) anthropogenic activity affects the size of its wetland habitat, the chemical properties of the water in which it lives, the success of other organisms (e.g., competitors, parasites, predators, and prey), and so forth. Sometimes the trouble is relatively localized—say, runoff from a nearby farm (Chapter 9) or habitat fragmentation—but it could also be linked to larger issues such as global climate change (Chapter 14). Second, protection of organisms and natural systems can be accomplished by two distinct, if similarly named, approaches: conservation and preservation.
- Conservation. This strategy is predominantly driven by a desire to directly improve human quality of life. In other words, conservation is management of ecosystems and organisms so they can provide natural resources. Organisms and biodiversity are certainly protected—as the word suggests, conservationists seek to maintain systems in their current condition indefinitely—but growth of organisms is optimized to meet the needs of people. For instance, regulations on the rates of logging, fishing, and hunting are intended to maximize harvests and profit while conserving valued commodities in perpetuity. The designation and maintenance of open spaces, forests, and parks to provide necessary habitats and for recreational purposes also are conservation efforts. We will see examples of conservation of valuable resources in Chapter 12.
- Preservation. Unlike conservation, preservation seeks to protect natural systems because of their ecological importance and / or intrinsic value. The goal here, then, is not to directly enhance human standards of living but to allow ecosystems to flourish without any interference. Organisms are decidedly not viewed as resources. In principle, they are shielded from not just harvesting and the like but hiking, camping, and any anthropogenic presence or activity. To be a bit flip about it, picture a forest with a large KEEP OUT sign on its border and you will get the idea. Pure preservation is, as you might imagine, far more difficult to accomplish and is becoming more and more rare as the size and standards of living of the human population continue to increase. In fact, it is not uncommon for so-called preserves to simultaneously serve as both havens for organisms and areas for human enjoyment, recreation, and similar activities.
Examples of protected species
One could find many examples of conservation of terrestrial and aquatic organisms and systems around the world. Due to the limited amount of space in this book, we will consider just a small number that demonstrate some important principles and problems.
Giant panda. The case of the panda merits some consideration because of its many complexities and the difficult questions it raises. This organism has been on the brink of extinction for decades due to poaching and habitat destruction. Their very inefficient reproductive strategy, narrow niche, and other unhelpful traits make them particularly vulnerable. What pandas do have going for them is fantastic public relations and passionate advocates. It is safe to say that people tend to find these creatures irresistible, adorable, and worthy of financial and political support (Figure 6.17).
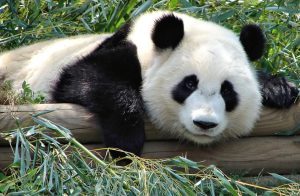
And support they get: billions of dollars have been spent in China (their only native habitat) and other countries to study, breed, display, and protect pandas. The population of wild pandas is very small—a few thousand individuals at best—and captive breeding programs have added some hundreds to that number.
This textbook is not the proper venue for a lengthy examination of the costs and benefits of panda conservation. In brief, there is hardly universal agreement that resources and efforts should be expended to save such an organism, although proponents make several arguments to support their point of view. First, all efforts to preserve biodiversity are valid, including those associated with pandas. Second, humans should fix this problem because they are largely responsible for its creation. Third, the panda is a powerful symbol for broad conservation and inspires protection of other endangered species. Finally, they are culturally and economically valuable. You are encouraged to read more about the complex financial aspects of panda conservation at the following link: The Value of Ecosystem Services from Giant Panda Reserves – PubMed . Some people, though, think panda conservation is a waste of money that could otherwise be spent on more ecologically relevant systems or even improving the conditions for impoverished humans. They argue that artificially propping up a poorly adapted organism is ultimately doomed to fail. Furthermore, recent research indicates that efforts to protect pandas in the wild have led to losses of large predators from the same habitats, including leopards, snow leopards, and wolves[17]. Importantly, data support the contention that focusing on a single species is likely to be less broadly successful than working to protect entire ecosystems. Another sticky yet crucial question is raised by the debate over pandas: is it appropriate for any species to receive a disproportionate share of attention (and help) because they are so aesthetically and emotionally appealing while organisms that are not so adorable (or, even subjectively ugly to humans) are ignored? In other words, in decision making about resource allocation and conservation efforts, what relative weight should be given to science, ethics, and popular opinion?
Aye-Aye[18]. Unlike the giant panda, this small, nocturnal mammal living on the Island of Madagascar (off southeast Africa) is decidedly not considered to be aesthetically (or otherwise) appealing by most people and has not garnered an enormous following of protectors and supporters (Figure 6.18).
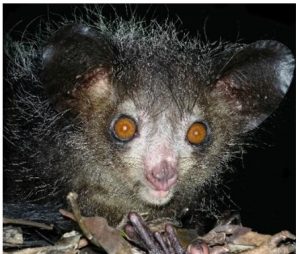
Should it be protected with rigor? The aye-aye has roles to play in its ecosystem, just like its more attractive animal kin.
Gray wolf [19]. This large predator once roamed through most of the lower U.S. states in numbers that approached hundreds of thousands of individuals (Figure 6.19). As human population density increased, so did conflicts between people and wolves: farmers, ranchers, parents of small children, tourists, and others sought to eliminate wolves to protect their various interests.
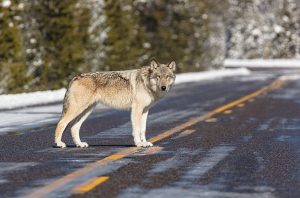
Starting in the late 1800s, these animals were hunted, poisoned, and driven out of nearly all their former ranges. Among the areas that saw populations drop to near zero by the 1920s was Yellowstone National Park in Montana, Idaho, and Wyoming (U.S.A.), and we will focus our attention on this important example. The complicated story of wolves in Yellowstone is rife with science, politics, advocacy, and competing interests. Even as some people did all they could to kill off these potentially threatening animals, others worked to protect them. In the early 1970s the gray wolf was added to the endangered species list, but its status was debated and changed during the decades that followed. The recovery plan for this organism included unsurprising protections against hunting as well as a controversial effort to re-introduce it into the park.
After many years of passionate debate and legal wrangling, a handful of wolves were moved from Canada into Yellowstone in the middle 1990s. In the following decades, the populations expanded, and the arguments between pro- and anti-restoration camps raged on. Keeping things brief, as the number of wolves increased in the 1990s, 2000s, and beyond, they were taken off and re-added to the endangered species list in the three relevant U.S. states several times. Data indicate the population has grown to a few hundred individuals, a level scientists consider to be healthy under the complex circumstances. But the issue continues to divide conservationists from ranchers and others, with scientists stuck somewhere in the middle trying to provide objective assessments of the effects of wolves on other predators (like coyotes) and prey (like elk).
Holmgren milk-vetch[20]. Our final example is a plant found in specialized environments in Utah and Arizona (U. S.). It is likely not one that is known to you, and the details of its history and threats to it are not necessary for our purposes. It is included here simply as a reminder that the list of endangered species includes not just animals, but also plants, fungi, and others (Figure 6.20).
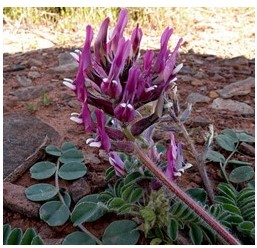
What about resuscitation of extinct organisms?
As our understanding of genetics grows, additional strategies to protect endangered species, or even bring back some that are lost, may become available. However, considering what we know now, extinction is a permanent condition, one that cannot be reversed through methods that likely will remain in the realm of fiction. Consult Box 6.5 for a bit more about this topic.
Box 6.5. Could we bring back dinosaurs?
The short answer to this question raised in multiple books and films is: sorry, no. Why not? First, DNA does not persist for more than tens of thousands of years, not even close to the 65 million (or more) that have elapsed since dinosaurs went extinct. In other words, the genetic information needed to pull off this nifty trick is gone. Second, even if we could clone dinosaurs, the likelihood they would survive is practically nil. Think like an environmental scientist: atmospheric chemistry (including the relative proportion of gases like dioxygen and carbon dioxide) is quite different today than it was back in the Jurassic Period. The properties of the solar radiation striking the planet’s surface have changed as well. Third, most of the plants and animals they ate are extinct today. While we are at it, many of the microorganisms that interacted with dinosaurs also are gone, meaning that digestion, immunity, and decomposition of their waste would likely be seriously hampered. Arguably, it is best that dinosaurs are relegated to history in any case—imagine the environmental changes we would experience if they came back.
Are we causing another mass extinction? As noted earlier, Earth’s biosphere has been affected by at least five previous periods of widespread extinction during the past 600 million years. Much evidence indicates that human activity is currently driving a sixth. This time around, though, the pace of loss is hundreds or thousands of times faster than we would expect under natural conditions. Researchers estimate that at least 400 vertebrates, including reptiles, amphibians, birds, and mammals, have gone extinct in the past century—losses that likely would have taken 10,000 years in the absence of humans; many invertebrates and plants have disappeared as well [21]. Projections of future human expansion during the coming century (Chapter 8) suggest that extinctions will continue at an ever-increasing rate in the future.
6.4. WHAT ARE THE BENEFITS OF BIODIVERSITY?
Before we close this chapter, we should address a lingering question: when it comes to biodiversity, is more always preferable to less? As usual, the answer depends on who you ask and under what circumstances you pose the question.
6.4.1. Conflicts can arise over it
We often face choices that pit human development against the protection of ecosystems and biodiversity. The economic and cultural benefits of, say, a new shopping mall, apartment complex, park, or farm must be compared to the value of maintaining a unique ecosystem that is one of the few remaining habitats of an endangered butterfly species. Priorities vary, as is well known.
6.4.2. Preserve it?
Analyses of the costs and benefits of preserving biodiversity, as well as the conflicts that tend to arise in these cases, are informed by both subjective, values-based reasons as well as objective, science-based evidence.
Subjective reasoning
We have noted before that the focus of this book is environmental science, not environmentalism. So, although we will acknowledge the passionate beliefs people hold about biodiversity, we will not be overly swayed by them. Instead, we will approach the problem as scientists.
Objective reasoning
The causes and relevance of biodiversity have been studied, but we still have much to learn about them. Two of the many questions we ask are briefly discussed here.
Does high genetic diversity provide benefits? We saw earlier that a single population with a large and diverse gene pool is more likely to adapt to and survive environmental change than one lacking such an advantage. Although it can help the affected species, is this diversity always beneficial to humans? If the organism is valuable to us (e.g., for economic, medical, environmental, or aesthetic reasons), we clearly appreciate genetic diversity. However, when it increases the fitness of disease-causing bacteria or crop-eating pests, outcomes we noted earlier, high genetic diversity is problematic and dangerous.
Is high species richness always best? This question may be more nuanced and controversial than you expect. Briefly, its answer is affected by many factors, including the type of ecosystem under consideration.
Artificially maintained environments
High species richness is often antithetical to accomplishing the goals of these systems. On a farm, for example, low biodiversity is generally preferable. As we will see in great detail in Chapter 9, food is usually produced in ecosystems manipulated by humans to maximize the growth of only one or a few organisms. In other words, high species richness is detrimental, and many steps are taken to reduce it. Public parks, golf courses, lawns, baseball fields, sports fisheries, and the like similarly strive to encourage the growth of a few desired organisms, that is, biodiversity in those places is intentionally kept low.
Natural environments
A widely held assumption is that high species richness is necessary to ensure the on-going health and success of all ecosystems on Earth. The larger the number of different species present, the argument goes, the more stable and able to withstand environmental change a system will be. High biodiversity is seen as a kind of insurance policy, if you will, analogous to high genetic richness within a single population. However, some ecologists question this view. They point out that since high biodiversity results in increased specialization and narrow niches, it could actually increase a system’s vulnerability to change. How could this be? Keep in mind that ecosystems with low diversity are dominated by a relatively small number of species that each occupy a broad niche. In other words, the functions active in them are carried out by generalists. In principle, such an organism would have an easier time adapting to change than would a specialist because the former already operates under a wider range of conditions than does the latter. So, even subtle alterations in average temperature, water availability, and prey identity have the potential to imperil many specialists. The same changes would also exert pressure on generalists, but they are more likely to endure and continue to dominate. Proponents of this idea note that, although environmental stability tends to induce higher species richness (as we learned above), the reverse is not necessarily true. Keep in mind that this logic should not be confused with advocacy to induce extinctions or otherwise reduce the number of different species on Earth! It is simply an objective evaluation of the science, one that is certainly not universally held. In any case, high biodiversity is a feature we value for multiple reasons, and declines in it would be taken as a sign of increased stress. Furthermore, although we know it can indicate healthy ecosystems, there is a great deal about the role and importance of biodiversity that has yet to be learned.
THE CHAPTER ESSENCE IN BRIEF [22]
However expressed, biodiversity is related to the number of species present in a defined space. Many natural and anthropogenic activities affect biodiversity. Two particularly important phenomena, biological evolution and extinction, can be understood as opposing forces that increase and decrease biodiversity, respectively.
Think about it some more…[23]
What do you think is the most useful way to express biodiversity?
How is the rise of a population of antibiotic-resistant bacteria a good example of evolution?
Is the well-known phrase “survival of the fittest” always consistent with evolutionary theory? If not, why not?
Are organisms that look and act like each other necessarily close relatives? Why or why not?
Why are small populations at elevated risk of extinction?
If extinction can be a natural process, why should we worry about it?
- Locey, KJ and Lennon, JT. 2016. Scaling laws predict global microbial diversity. Proceedings of the national academy of sciences. 113:5970–5975 ↵
- Tittensor, MC, Adl S, Simpson AGB, Worm B. 2011. How Many Species Are There on Earth and in the Ocean? PLoS Biol 9(8): e1001127. doi:10.1371/journal.pbio.1001127. CC BY ↵
- Thewissen, JGM., Cooper, LN, George, JC et al. Evo Edu Outreach. 2009. 2:272. doi:10.1007/s12052-009-0135-2. CC BY ↵
- Endler. JA. 1980. Natural Selection on Color Patterns in Poecilia reticulate. Evolution. 34:76-91. Public domain. https://pubmed.ncbi.nlm.nih.gov/28563214/ ↵
- Wayne RK , Nash WG , O'Brien SJ. 1987. Evolution of the Canidae. II. Divergence from the primitive carnivore karyotype. 44:134-141. Public domain. https://pubmed.ncbi.nlm.nih.gov/3568762/ Cytogenetics and Cell Genetics ↵
- Alvarez G, Ceballos FC, Quinteiro C (2009) The Role of Inbreeding in the Extinction of a European Royal Dynasty. PLoS ONE 4(4): e5174. CC BY. https://doi.org/10.1371/journal.pone.0005174. https://journals.plos.org/plosone/article?id=10.1371/journal.pone.0005174 ↵
- U.S. EPA. Endangered species. 2019. https://www.epa.gov/endangered-species/learn-more-about-threatened-and-endangered-species ↵
- National Parks Service. 2016. https://www.nps.gov/glba/blogs/a-keystone-species-the-sea-otter-colonizes-glacier-bay.htm ↵
- Fish and Wildlife Service. 2017. https://www.nps.gov/articles/what-is-white-nose-syndrome.htm ↵
- U.S. EPA. 2020. Watershed Academy. cfpub.epa.gov/watertrain/moduleFrame.cfm?parent_object_id=540 ↵
- U.S. Fish and Wildlife Service. ecos.fws.gov/ecp/species/4080 ↵
- Fralish, J.S. 2004. The Keystone Role of Oak and Hickory in the Central Hardwood Forest. U.S. Forest Service. fs.usda.gov/treesearch/pubs/6500 ↵
- U.S. National Institutes of Health. dtp.cancer.gov/timeline/flash/success_stories/S2_Taxol.htm. ↵
- Fish and Wildlife Service. 2023. Endangered species. www.fws.gov/program/endangered-species ↵
- Association of zoos and aquariums memorandum of understanding. U.S. Fish and Wildlife Service. www.fws.gov/program/endangered-species/aza-memorandum-of-understanding ↵
- U.S. Fish and Wildlife Service. International affairs. www.federalgrants.com/Wildlife-Without-Borders-Africa-Program-53680.html ↵
- Li, S., McShea, W.J., Wang, D. et al. 2020. Retreat of large carnivores across the giant panda distribution range. Nature Ecology and Evolution 4:1327–1331 ↵
- Fish and Wildlife service. ecos.fws.gov/ecp/species/7643 ↵
- Data about wolves are derived from the following two sources. (1) Fish and Wildlife Service. 2020. Endangered and Threatened Wildlife and Plants; Removing the Gray Wolf (Canis lupus). From the List of Endangered and Threatened Wildlife. www.federalregister.gov/documents/2020/11/03/2020-24171/endangered-and-threatened-wildlife-and-plants-removing-the-gray-wolf-canis-lupus-from-the-list-of (2) Wolf restoration. National Parks Service. nps.gov/yell/learn/nature/wolf-restoration.htm ↵
- U.S. Fish and Wildlife Service. Environmental conservation online system, ECOS online. ecos.fws.gov/ecp/species/4590 ↵
- Cebellos, G., Ehrlich, P.R., Raven, P.H. 2020. Vertebrates on the brink as indicators of biological annihilation and the sixth mass extinction. Proceedings of the National Academy of Science of the United States of America. doi.org/10.1073/pnas.1922686117. CC BY ↵
- As you will find throughout this book, here is very succinct summary of the major themes and conclusions of Chapter 6 distilled down to a few sentences and fit to be printed on a t-shirt or posted to social media. ↵
- These questions should not be viewed as an exhaustive review of this chapter; rather, they are intended to provide a starting point for you to contemplate and synthesize some important ideas you have learned so far. ↵
Refers to organisms that are too small to be seen without the aid of some kind of magnifying lens (e.g., a microscope). Includes bacteria, protozoa, certain forms of algae and fungi, and viruses. See Chapters 1 and 5 for more.
A cell that consists of discreet, membrane-enclosed subcellular structures like nucleus, chloroplast, ribosomes, and mitochondria. Contrast with prokaryotic cell. Also known simply as 'eukaryote'. See Chapter 3 for more.
The role an organism plays in its habitat. A niche can be narrow, that is, organisms occupying it have very specific needs and activities. Slight changes to the conditions in an ecosystem could devastate such organisms. On the other hand, a niche can be broad, meaning that organisms in it can use a wider variety of strategies to meet their needs. In this latter case, organisms are more likely to survive changes to environmental conditions. Compare to habitat. See Chapters 5 and 6 for more details.
Refers to all the information that can be used to copy cells. See Chapter 6 for more.
Through the use of one of several techniques, the DNA sequence of an organism can be altered. For example, cross breeding of related species can produce offspring with desired traits; genetic engineering that involves direct manipulation of DNA sequences is a modern way to alter the genome of an organism. Genetically modified organisms are often used in agriculture to increase food production, but they are controversial. See Chapter 9 for more.
An adjective referring to an organism that lacks membrane-bound organelles. See Chapter 3 for more.
A type of reproduction in which a individual can reproduce without a mate. Bacteria and protozoa, for example, reproduce this way: a single cell divides into two identical cells. Contrast this strategy with sexual reproduction. See Chapter 6 for more.
In ecology, a group of organisms that are all members of the same species. See Chapter 5 for details.
A scientific notion that has developed after many experiments; often, several generations of scientists have tested and refined such a notion before it is elevated to the status of theory. It is reasonable to view a theory as a conclusion that has withstood many attempts to discredit it. Note that “theory” is used differently in science than it is in everyday life. In the former, it is taken very seriously, whereas in the latter it indicates an idea that has little or no evidence to support it. Even well-supported theories could be modified with continued study, but a lot of new data would need to come to light in order to discredit one of them. Compare to conclusion and law. See Chapter 2 for more.
A proposed explanation for an observed phenomenon. See Chapter 2 for more.
An adjective that refers to something that originates from within an organism. For example, endogenous errors in DNA are not caused by external agents but by some forces associated with the body. See Chapter 6 for a little more.
The remains of an ancient organism. Such remains can take many forms, including impressions in rocks or chemical remnants that were transformed into fuels such as coal, oil, and gas. See Chapters 3 and 10 for more.
More formally, 'anaerobic organisms.' They do not require dioxygen gas; in fact, many anaerobes are poisoned by O2. See Chapter 1, especially Box 1.1, for more.
An organism that uses the sun as its energy source. See Chapter 5 for more.
A process carried out by certain organisms (plants, algae, and some bacteria) in which the energy of the sun is used to drive the fixation of carbon. See Chapters 4 and 5 for more details.
In ecology, a general term referring to two or more organisms living together. Symbiotic and symbiotically are related words. See Chapter 5 for more.
An important subunit or organelle in eukaryotic cells that converts energy into usable forms for a cell. See Chapter 3 for more.
More formally, 'aerobic organisms', they require dioxygen gas for survival. See Chapter 1, especially Box 1.1, for more.
Rivalry among members of a species, that is, those in the same population. Compare to interspecific competition. See Chapter 5 for more.
In ecology, the sequential replacement of dominant species in an area; it is mechanism by which an ecological community comes into being. See Chapter 5 for details.
In ecology, an organism that is not native to an area in which it currently lives. See Chapters 5 and 6 for more.
In ecology, exotic species that outcompete native organisms. Often, invasives can take over an area. See Chapters 5 and 6 for more.
In ecosystems, a group of organisms that all feed the same number of steps away from the original source of energy. For example, plants are all at the first trophic level because they get their energy directly from the sun, whereas deer and other herbivores are at the second trophic level because they get their energy from eating organisms at the first trophic level. Consult Chapter 5 for more details.
One of the processes carried out by organisms in an ecosystem. Important functions include C fixation, decomposition, and consumption. See Chapter 5 for details.
In ecology, refers to the specific identity of organisms present in an ecosystem. This property varies widely among ecosystems. See Chapter 5 for details.
This is animal with a backbone (e.g., humans, snakes, cats, mice, barracuda). Compare to invertebrate.