4 Earth’s Environments, Cycles, and Energy
Jason Kelsey
In Chapter 1 we were introduced to topics studied by environmental scientists, including factors influencing the availability of materials on Earth. There is good reason for our interest in this area: both human and natural systems must have ready access to necessary nutrients if they are to function. Here we will examine more details about the storage, movement, and recycling of some vital materials. We will also take our first look at the fundamentals of energy, a topic important to natural and human systems and one that will appear again in upcoming chapters.
Key concepts
After reading Chapter 4, you should understand the following:
- The characteristics of Earth’s four spheres as identified and studied by environmental scientists
- How Earth is a system that is closed with respect to materials and open with respect to energy
- The chemical, physical, and biological processes responsible for the movement and recycling of Earth’s atoms among its four spheres
- The major reservoirs and pathways of the carbon, nitrogen, and phosphorus cycles and why these cycles are important to both Earth’s systems and environmental scientists
- How both insufficient and excess nutrients can disrupt a system
- The reservoirs and pathways of the water cycle
- How atoms in the lithosphere are moved and recycled through the tectonic and rock cycles
- The sources of energy for Earth’s systems
- How energy is converted from high- to low-grade forms
- How the first and second laws of thermodynamics limit all of Earth’s processes and systems
Chapter contents
4.1. Earth’s Spheres
4.2. Cycling of Materials
4.3. Movement of Energy
The Chapter Essence in Brief
4.1. EARTH’S SPHERES
Materials move within and among Earth’s many systems. Before proceeding with a detailed study of cycles, we will look at the four basic zones or spheres within which materials of interest to us are found.
4.1.1. Atmosphere
Put very succinctly, this sphere is the envelope of gases surrounding the Earth. It extends from the surface outward, up to approximately 10,000 km into space in some places. The atmosphere is subdivided into several horizontal layers (also known as spheres), of which only the lower two will receive much attention from us. The altitudes listed are approximate[1]. See Figure 4.1 for a schematic diagram of the atmosphere.
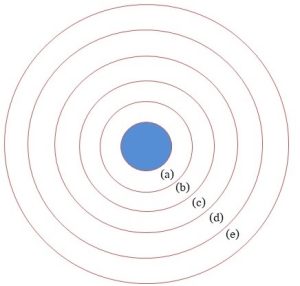
(a) Troposphere (b) Stratosphere
(c) Mesosphere (d) Thermosphere (e) Exosphere
Note: the ionosphere overlaps spheres c – e and is not shown. See main text for more details. Kelsey, CC BY-NC-SA.
Troposphere: 0 – 15 km
This is the part of the atmosphere with which we have direct contact and the place in which weather events occur. The bulk of atmospheric gases are found here, including dinitrogen, N2 (makes up about 78 %), dioxygen, O2 (21 %), argon, Ar, and carbon dioxide, CO2 (together, these last two make up a little less than 1 %), and many other gases present in very small amounts. Note that the list in the previous sentence reflects the current atmosphere. A lot of evidence found in rocks and ice indicates that the composition of tropospheric gases has changed throughout history. Notably, the O2 that organisms like us need for survival only appeared about 2.5 billion years ago (about 2 billion years after Earth was formed, as we saw in Chapters 1 and 3). So, the troposphere is a very dynamic layer, one which interacts with and is influenced by living and non-living systems at the surface. The outer limit of this zone, that is, the boundary between it and the next sphere, is called the tropopause. We will see more about the troposphere when we study air pollution in Chapter 14.
Stratosphere: 15 – 50 km
Unlike the troposphere, which gets colder as one travels outward, here temperature increases with altitude. This difference is due in part to chemical changes. Most importantly for our study, atmospheric ozone (O3) is concentrated in this layer (Chapter 14).
Mesosphere: 50 – 90 km
This zone is important to life on Earth because it is where the bulk of meteorites entering the atmosphere burn up before they strike the surface.
Thermosphere: 90 – 500 (up to 1000) km
This is often thought of as the boundary between Earth’s atmosphere and outer space.
Exosphere: from the top of the thermosphere (500 – 1000 km) to 10,000 km
Human-launched satellites orbit within this zone.
Ionosphere: 50 – 1000 km
This zone overlaps the mesosphere, thermosphere, and exosphere. It is characterized by its charged nature—radiation from the sun generates a lot of ions and electrons (more about these particles is presented below). These particles reflect radio waves, enabling long-distance communication.
4.1.2. Hydrosphere
Formally, the hydrosphere contains all the water on Earth. Now, as will become increasingly clear, water is not found exclusively in this area. In fact, none of these definitions should be taken as inflexible delineations because the four spheres interact quite a bit. For now, though, hydrosphere is a convenient and useful term, one that we will explore in detail shortly.
4.1.3. Lithosphere
As we learned in Chapter 3, the lithosphere is the brittle, outer-most layer of the solid Earth and is comprised of the crust and the upper mantle. The shape of the planet’s surface features, as well as the cycling of its materials, are linked to the movement of lithospheric plates across the top of the asthenosphere (see Figures 3.1 and 3.2 in the previous chapter).
4.1.4. Biosphere
The fourth sphere simply refers to all living matter. Many subdivisions can be employed to define and organize the vast diversity of organisms found on this planet. For our purposes, though, only broad categories are necessary to understand the ways this sphere interacts with the others and the important roles it plays in shaping Earth’s systems.
Land and water organisms
Members of the biosphere are found in many different environments. We will explore this distribution in more detail in upcoming chapters, but for now you should recognize two major categories. Terrestrial organisms live largely on land, and include plants such as trees, microorganisms such as bacteria, and animals such as rats, lizards, tigers, and humans. Aquatic organisms live in water, so whales, sharks, lobsters, jellyfish, algae, and non-terrestrial bacteria are grouped here. We can further divide this second group into those that live in freshwater environments like rivers and lakes and those that are found in saltwater, or marine, environments such as oceans.
Microscopic organisms (review Chapter 3)
Microbes are things too small to be viewed with the naked eye—a magnifier, like a microscope, is required to see them. Despite their practical invisibility, living microbes, generally called microorganisms, are immensely important to the functioning of Earth’s natural systems. This very diverse group includes bacteria, protozoa, algae, and fungi (although not exactly alive, we also categorize viruses here).
Macroscopic organisms
Organisms in this group are large enough to be viewed without the aid of a microscope. These appear to dominate Earth’s systems, but they are dependent on microorganisms for their survival.
Plants. An exhaustive description of these organisms is not necessary for this book, but a short overview of their most important characteristics will help us to understand the roles they play. Plants are multicellular, eukaryotic (Chapter 3), immobile organisms that extract water and many of the nutrients they need from the soil in which they are rooted. They are distinguished from animals, the other major macroscopic organisms on our list, in large part by their ability to use the sun for energy and CO2 in the atmosphere as their source of carbon (more in the discussion of the carbon cycle, shortly). In so doing, plants often form the foundation for land-based ecological systems. We will return to a discussion of the ways different kinds of organisms obtain necessary nutrients, including energy and carbon, as well as interactions among members of the biosphere, in Chapter 5.
Animals. These are also eukaryotic and multicellular. Unlike plants, though, animals tend to be mobile and use neither the sun nor CO2 directly to meet their needs. Instead, they depend on plants (or plant-like organisms) for biologically useable forms of energy and carbon, that is, animals consume what plants produce. We will revisit these ideas later (notably, Chapters 5 and 9)
4.2. CYCLING OF MATERIALS
As we learned in Chapter 1, the assumption that Earth is closed with respect to materials is fundamental to our study of environmental science. Just as critical, though, is the fact that Earth is open with respect to energy.
We will learn more about the differences between materials and energy later in this chapter, but for now it is important to realize that materials are recycled, whereas energy is not (Figure 4.2 provides a basic model to distinguishes their behavior).
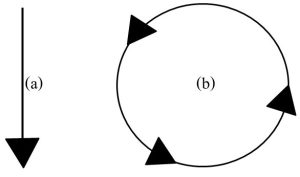
4.2.1. A few words about chemistry
An exploration of Earth’s materials requires a brief introduction to some essential terms and principles.
Chemistry
This is the science that investigates matter and changes it can undergo (informally, matter is just stuff—physical entities that take up space). Of particular interest are the fundamental composition and properties of the building blocks of everything in the universe and the ways these building blocks interact with (also react with; see below) each other. Often, chemists study what happens to matter on a very small scale.
Atoms
These are the tiny building blocks of all matter, the smallest units into which any element (see below) can be divided.
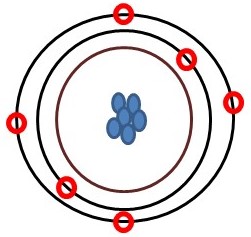
An atom is made up of even smaller components, subatomic particles such as protons and neutrons in its nucleus and electrons in clouds in its exterior. A neutral atom had an equal number of protons and electrons. Figure 4.3 is a simplified model of an atom.
Ions
An atom has the same number of positively charged particles (protons) as negatively charged ones (electrons). Atoms, therefore, have no net charge. For various reasons, the number of electrons in an atom may change, though, yielding an entity called an ion. Atoms are said to be reduced when they gain electrons and become negatively charged, whereas those that lose electrons are oxidized to take on a positive charge. Keep in mind that ions behave differently than neutral atoms, generally dissolving more easily in water and binding to other charged particles.
Element
This term refers to a substance that cannot be broken down into simpler units by ordinary means. It is related to a previous term, above, in that atoms with the same basic chemical properties are placed into categories called elements. As of 2022, there were 118 known elements, although some elements are more relevant to us, as both living beings and environmental scientists, than others. Note that the terms “atoms” and “elements” are sometimes used interchangeably, but they do not refer to the same things. One casual way to think about it: atoms with the same chemical properties are members of the same club, that is, they are grouped together as one element. For example, all the atoms in the universe having 6 protons in their nucleus are classified as the element carbon (C).
Bond
Individual atoms can be connected or attached to each other through various attractive forces or bonds. We use capital letters to represent individual atoms and short lines to represent bonds. For example, H – H is shorthand for a molecule made of two hydrogen atoms connected by a single bond, and O = O shows two oxygen atoms held together via a double bond.
Molecule
When two or more atoms undergo bonding, they form a molecule. For example, under the right conditions 2 hydrogen atoms and 1 oxygen atom can be brought together to form a water molecule, H2O. See Figure 4.4.

Compound
This is a molecule with more than one type of element in it. So, O2 is classified as a molecule but not a compound, whereas H2O is both a molecule and a compound.
Reaction
Two or more atoms brought close together can undergo changes as a result of chemical reactions. For example, atoms and electrons within molecules can be added, removed, or rearranged, and existing bonds can be broken and new bonds formed. Some of the energy released during certain reactions can be captured and used to power both living and non-living systems.
4.2.2. Cycling of elements
Reservoirs and pathways of elements
Environmental scientists are interested in both the storage and movement of elements within and among the four spheres we described near the beginning of this chapter. Since atoms can be tracked through living and non-living reservoirs, we use the term biogeochemical cycles to indicate the scope of our work.
Recycling and reuse
Since Earth is closed with respect to materials, it does not gain or lose appreciable amounts of any atoms—what is present now has been present throughout history. Consider what this means: the atoms in its system must be used and reused over and over again. For example, an individual carbon atom has been a part of many, many different objects and systems since Earth was formed. That same C could have been a component of a gas in the atmosphere, then moved into the biosphere to form part of the tissue of an organism, then on to the lithosphere where it might have been incorporated into a rock and stored for millions of years. Eventually, the decomposition of the rock could have released that atom into water, where it combined with other atoms to build the shell of an oyster. There is a staggering number of possibilities. Keep in mind that, although atoms are reused, they often undergo changes in chemical form as they travel from one system to another. It will not likely be a surprise to you that a C atom in atmospheric carbon dioxide is connected to different atoms, via different kinds of bonds, than that same C atom in, say, a plant leaf. In fact, the C atoms in those two examples are different in one fundamental way: as part of the leaf it is said to be organic, whereas in the CO2 gas it is in an inorganic form (see Box 4.1 for more).
Box 4.1. What is the difference between organic and inorganic?
The word “organic” appears in many places these days. For example, it can be used in reference to certain kinds of food and clothing to suggest something about the methods used to produce them. Often it is viewed as synonymous with “natural”, “pure”, and “good”, particularly in marketing to health-conscious consumers. To a chemist, though, the term refers to a simple set of measurable criteria employed to evaluate substances. A chemical compound is classified as organic if 1) at least one carbon atom is present in it and 2) there is at least one C-H bond present, that is, at least one carbon is attached to at least one hydrogen atom. The term inorganic refers to a compound that does not meet both criteria required to be called organic. So, a compound that lacks any carbon atoms or has one C atom but no C-H bonds is inorganic. Let’s compare the following three examples: C6H12O6 (a molecule with 6 carbons, 12 hydrogens, and 6 oxygens), CO2, and H2O. The first is organic because it has at least one carbon and one carbon-hydrogen bond, but the other two are inorganic as CO2 does not have any C-H bonds and H2O lacks C. Note that characteristics such as source, appearance, and nutritional value are irrelevant here. Both classes of compounds can be produced by natural forces and both can be synthesized under laboratory conditions. We will use these terms to convey information about chemistry, not to express value judgments.
Importance of chemical form
As will become clear, both the location and chemical form of an element are equally important to us. It is vital to understand the distinction between the mere presence of an element in a sphere and the relative usability of that element to organisms (often referred to as bioavailability). In other words, an element that is abundant in an area will not necessarily be utilized by living things. Since only certain chemical forms of elements can be assimilated by members of the biosphere, processes that affect bioavailability of individual atoms, either by increasing or decreasing it, are of great concern.
The details vary from element to element, but cycles have some basic processes in common.
Fixation. Broadly applied, this term refers to chemical transformations that increase the bioavailability of an atom. Not all organisms can carry out fixation, so those that can are critical to the success of ecological systems (Chapter 5). Fixation tends to involve the production of relatively large molecules from the transformations and re-combinations of smaller ones.
Assimilation. Here, biologically available forms of elements are absorbed by members of the biosphere and used to construct various cells and tissues. An atom in one organism cannot be used by other organisms until it is excreted or moved via consumption (like it sounds, eaten by another; more below).
Decomposition. This general term can be applied whenever a large, complex object is reduced to smaller, simpler products. For example, the frustration brought on by a misbehaving computer could be alleviated, albeit temporarily, if one uses a hammer to smash the offending machine into tiny pieces—physical decomposition or disintegration would be the result (along with the need to spend money on a new CPU). A related, but fundamentally different kind of breakdown occurs when large complex molecules are chemically decomposed. In this case, bonds are broken by biological or non-biological means and individual atoms or smaller molecules are released. As we will see, fungi and some bacteria are responsible for a great deal of chemical decomposition. Although reality is a little more complicated, it is useful to imagine this process as essentially the opposite of fixation. Keep in mind our cycling context: decomposition frees atoms, allowing them to undergo any number of transformations, including fixation. Without this process, waste and remains of dead organisms (grouped together as dead organic matter) would pile up on Earth.
Respiration. This process enables organisms to gain energy from the food they eat. Note that decomposition and respiration are closely related because, when chemical compounds in food are broken down, some of the released energy can be captured by the organism and converted into a form that is biologically available. Often, that energy is stored inside the cells of organisms until needed. Realize that not all organisms use the same strategy to obtain energy. For example, aerobic organisms like humans need O2 gas to keep respiration going. Anaerobic organisms (certain bacteria and fungi) use molecules other than O2. We will see more about the ways organisms get nutrients in Chapter 5.
Excretion. Nutrients that have been assimilated will ultimately be broken down and released in waste such as urine and feces. Since excrement generally contains forms of elements that are poisonous to the organism producing it, the ability to effectively release it is crucial for survival. Interestingly, though, toxicity and usefulness are rather relative: waste to one organism can be food for another. To decomposers, your unwanted products make up a nutrient-rich meal. Insects feasting on a pile of animal dung similarly thrive on what you (likely) consider unpalatable and unhealthy. In addition to feeding themselves, these feces-eating organisms also make an essential contribution to the recycling of Earth’s materials because they free up elements that would otherwise remain unavailable.
Weathering and erosion. These two processes are often grouped together, although they are technically distinct. Weathering is a general term that refers to the breakdown and change to Earth’s surface features brought about by forces such as rain, wind, and ice. Erosion is responsible for the transport of weathering products—bits and pieces of rocks, if you will—to other locations (Chapter 3). Think back to the hypothetical C atom we imagined earlier for a minute: weathering and erosion would have been responsible for its release from the rock and movement to the ocean.
Diffusion. This physical process moves materials from locations in which they are relatively abundant, referred to areas of high concentration, into areas in which they are present in low concentration. Imagine what would happen if you added a few drops of grape juice to a clear glass of water. At first you would see obvious purple clouds floating near the top of the container. In a short period, though, differences in color would disappear as the juice spread out evenly throughout the system. In all likelihood, the entire volume of water would eventually become the same light purple color due to diffusion of the grape juice from its point of highest concentration—where it entered the water—to those with lower concentrations. Similar processes are active on much larger scales and profoundly affect the distribution of elements among systems. The flow of CO2 between the atmosphere and the hydrosphere is just one important instance in which diffusion has important consequences for organisms living in water as well as global climate (more about these later).
Combustion. Objects can ignite and burn as a result of both natural and human activity. In this chapter we will focus more on the latter than the former because of its potential to quickly change the distribution of large amounts of material in a relatively short period of time. The combustion of fuels such as coal and oil along with the use of fire to clear large forested regions are two notable and important examples of human activities responsible for the conversions of solids to gases and the movement of large amounts of materials into the atmosphere. We will return to these topics in detail in Chapters 10, 12, and 14.
Limits of tolerance
How much of a substance is present—often referred to as its dose or concentration—will determine if that substance is beneficial or detrimental. Put another way, there is an optimal level for all materials, including chemical elements. Too little and too much of an essential nutrient can harm a system. If insufficient amounts of a vital material are available to organisms, they will not be able to properly grow and reproduce. On the other hand, an excess of that same material can bring about drastic and unwelcome changes. We will see what can happen if levels of tolerance are exceeded shortly.
Rate of movement
The time required to move and recycle different chemical elements varies. Because gaseous forms of elements can travel quickly among reservoirs, elements that do not spend much time in the atmosphere tend to move more slowly than those that do. Also, biological processes generally are faster than non-biological ones. The elements we will discuss here have dramatically different residence times in different reservoirs, ranging from days (some atoms in the atmosphere) to hundreds of years (some atoms in the hydrosphere) to hundreds of millions of years (some atoms in the lithosphere).
Three important examples: carbon, nitrogen, and phosphorus
Of the many known elements, we will focus on the movements of just carbon, nitrogen, and phosphorus because of the important roles they play and the useful examples they provide.
We need carbon
Why is carbon on our short list? The first part of the answer is its value to the members of the biosphere. Organisms must have access to sufficient biologically available C atoms or they will not be able to construct the organic compounds that make up their cells and tissues. Carbon is simply crucial for the survival of all living things. Carbon-containing compounds are also important sources of energy for the vast majority of organisms. Secondly, it is central to many of the environmental concerns we will explore in upcoming chapters, including ecosystems, agriculture, energy resources, waste management, and air pollution (Chapters 5, 9, 10, 13, and 14, respectively).
Carbon is versatile
Its chemical properties allow C atoms to bind in many different ways to a wide range of atoms. As a consequence, we see tremendous diversity within the biosphere, with organisms taking on a variety of physical forms. But carbon does not stop there—it is central to the structure of many non-living things as well, from natural objects such as diamonds, coal, and oil to synthetic polymers, building materials, drugs, industrial chemicals, and pesticides. We could devote several textbooks to the wonders of carbon. Instead, though, we will focus on three important compounds in the carbon cycle.
C = O = C
- Chemistry. It is inorganic.
- Phase. It is a gas under most conditions on Earth (but solid dry ice when frozen).
- Sources. It is released as a product of aerobic respiration (e.g., each time animals like us exhale), from volcanoes, and as a product of the combustion of organic carbon compounds (e.g., wood, oil, coal). It can also be the result of chemical transformation of methane (see the next compound on this list).
- Biological availability. This form cannot be utilized directly to build cells and tissues. Carbon-fixing organisms like plants and algae, called producers, can convert it into glucose, a form that is usable to all organisms (see the third compound on this list). The actions of producers are essential to Earth’s consumers, including animals and many microorganisms, which cannot fix carbon. The relationship between producers and consumers is explored in more detail in Chapter 5.
- Reservoirs and fates. It is stored primarily in the atmosphere and hydrosphere, but some is also present in the lithosphere. As we have just seen, it can also be fixed by producers.
- Important pathways. Decomposition, respiration, and fixation are carried out by organisms and can cause rapid conversions among this and other forms. Combustion of organic carbon compounds found in materials such as wood, coal, oil, trash, and animal waste releases CO2 into the atmosphere, and diffusion can move it from the atmosphere into the hydrosphere (and back again).
Methane [CH4]
- Chemistry. It is organic.
- Phase. It is a gas under most conditions on Earth.
- Sources. It is released from anaerobic respiration and decomposition (e.g., certain bacteria), from some animals (e.g., cows) and as a product of combustion (as seen with carbon dioxide, above).
- Biological availability. Other than a small number of very specialized bacteria, organisms cannot use this form of C directly.
- Reservoirs and fates. It is stored primarily in the atmosphere and hydrosphere, but some is also present in the lithosphere. As noted, it can be used as a nutrient source by certain bacteria and some is converted to carbon dioxide.
- Important pathways. Anaerobic respiration (see above) and combustion are the two major pathways affecting the distribution of methane.
Glucose [C6H12O6]
- Chemistry. It is organic.
- Phase. It is typically solid.
- Source. Carbon-fixing organisms (see above) can convert carbon dioxide into glucose.
- Biological availability. This form of C can be used by all members of the biosphere as a basic starting point for the construction of many cell components such as fats, proteins, and carbohydrates.
- Reservoirs and fates. Carbohydrates like glucose are present in living organisms and the lithosphere. Since it is the preferred nutrient source for many organisms, glucose is converted to carbon dioxide by aerobic organisms or a gas such as methane by anaerobes. In other words, consumption and respiration lead to the release of unavailable, gaseous forms of carbon. Consult Box 4.2 for the story of what happens to carbon compounds that enter organisms.
- Important pathways. Fixation produces glucose, and decomposition and respiration break it down to smaller and less useable compounds like carbon dioxide. Consumption in the biosphere, as well as erosion and combustion, also affect the distribution of glucose and its precursors and products.
Box 4.2. Just what are we doing with that food we eat?
As we have learned, organisms, including humans, need to find biologically available forms of carbon to survive. To do that, of course, we eat food. But just what are we doing with glucose after we obtain it? Three fates are possible. First, when the bonds holding the molecule’s atoms together are broken, stored energy is released. Some of that energy is absorbed and used immediately, or later, to do work. The carbons that were in the glucose are released to the atmosphere as a product of our respiration. Second, some of the C atoms stay inside our bodies and are used as building blocks to construct new cells and tissues. Finally, we release some of the compounds in our waste. Those unwanted products still have stored energy and usable carbon in them, even if we can no longer use them. Keep in mind that somebody can use them, though: certain organisms live off excrement, extracting needed energy and other nutrients from it.
Carbon fixation is a crucial step in the cycle
There are a few mechanisms by which carbon dioxide is fixed into glucose, although photosynthesis is the most common (and likely the most familiar).
6CO2 + 6H2O → C6H12O6 + 6O2
Plants, algae, and other organisms can use the sun’s energy to produce one glucose from six carbon dioxide and six water molecules. This fixed carbon is then used by both producers and consumers in the biosphere. You should also notice the six oxygen molecules, O2, released during carbon fixation. That second compound is of no small importance to all of us aerobes on Earth: without photosynthesis, the atmosphere would not contain breathable oxygen (more in Chapter 1).
Carbon moves among reservoirs via pathways
As with all the materials we will consider, C atoms are distributed among Earth’s spheres as they travel along the pathways described above. Just as important as their location is their chemical form: remember that carbon dioxide may be abundant, but success of organisms depends on how much of it is converted to glucose. Figure 4.5 connects the reservoirs and pathways of the carbon cycle.
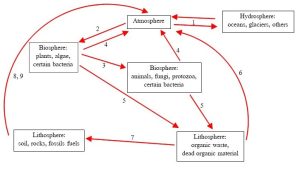
1. Diffusion moves CO2 between the atmosphere and hydrosphere. Note there is no change in chemistry of the C, just a change in its location.
2. Carbon fixation by producers converts CO2 in the atmosphere (or, in the case of aquatic systems, hydrosphere) to glucose. This moves C into the biosphere.
3. Fixed carbon moves within the biosphere from producers to consumers.
4. Respiration by all organisms moves CO2 (aerobes) and CH4 (anaerobes) back to the atmosphere.
5. Organic carbon in the waste and remains of living things can accumulate in the lithosphere.
6. Decomposition and respiration by fungi and certain bacteria convert some of the organic material in the lithosphere into CO2 (aerobes) and CH4 (anaerobes). The gases can move back to the atmosphere.
7. Some fraction of the organic material in the lithosphere escapes decomposition and is stored for an extended period in soils, rocks, and fossil fuels (more on fossil fuels can be found in Chapter 10).
8. Combustion of fossil fuels converts organic C in the lithosphere into CO2 and other gases.
9. Slow decomposition of some of the organic matter in the lithosphere converts organic C into CO2 (aerobes) and CH4 (anaerobes). Kelsey, CC BY-NC-SA.
We need nitrogen
Like carbon, nitrogen is essential to members of the biosphere: without biologically available forms of N, organisms cannot make proteins. Although less nitrogen is required than carbon, it is still vital to our survival. We will also see that nitrogen-containing compounds play important roles in our study of agriculture (Chapter 9), water pollution (Chapter 13), and air pollution (Chapter 14).
There are many forms of nitrogen
Nitrogen is a versatile element and can be found in different types of materials. For the sake of efficiency and simplicity, we will consider just four compounds here.
Dinitrogen [N2]
- Chemistry. It is inorganic (no C atoms are present).
- Phase. It is a gas under most conditions on Earth.
- Sources. Recall from the beginning of this chapter that N2 makes up about 78% of the gases in the atmosphere. It became so abundant during Earth’s long history due to several geologic processes. On today’s Earth, decomposition and combustion of organic material release a variety of N gases that can be converted to N2 in the atmosphere.
- Biological availability. This form of nitrogen cannot be used to build any biological structures. A specialized group of bacteria, collectively known as nitrogen fixers, can absorb dinitrogen and convert it into forms that are directly usable by microorganisms and plants.
- Reservoirs and fates. This form is found primarily in Earth’s atmosphere, although a little is present in water and soil. As noted above, it can be fixed under certain conditions. Nitrogen fixation is described in more detail below.
- Important pathways. Fixation, decomposition, respiration, and combustion all participate in the movement of N2.
Ammonium [NH4+] and Nitrate [NO3–]
- Chemistry. Both are inorganic.
- Phase. These ions usually are dissolved in water or bonded to soil solids. Note that the + indicates a lost electron and positive charge and the – means a gained electron and negative charge (ions are described above).
- Sources. Ammonium is produced from N2 by nitrogen-fixing bacteria, and nitrate is produced from ammonium by other bacteria. The two compounds can also be synthesized artificially and applied to soil in fertilizers. Decomposition of waste products and amino acids (the next N compound on our list) in the remains of organisms will yield them as well.
- Biological availability. Both can be used as a source of N by microorganisms and plants, but not by animals.
- Reservoirs and fates. These compounds are primarily found in soil and water. Microorganisms and plants can absorb and convert them into amino acids, the next N compound on our list. Under different conditions, denitrifying bacteria can convert ammonium and nitrate back into nitrogen gases such as N2.
- Important pathways. Fixation, decomposition, assimilation, excretion, and denitrification all play important roles in the cycling of ammonium and nitrate. Downward movement through soil in flowing water, known as leaching, and horizontal movement or runoff across the Earth’s surface will also carry these and other compounds (more about water movement is presented a bit later).
Amino acids [RCH(NH2)COOH]
- Chemistry. These are organic compounds (recall: at least one C bonded to at least one H). Note that the structure shown above is a generalized model of the twenty different amino acids commonly found in the biosphere. The R does not represent an atom of a particular element, rather it is used to indicate a variety of different possible atoms or groups of atoms that can be located in that place—each of the twenty has a different group in the R position.
- Phase. They are generally found in the solid phase.
- Sources. As noted above, the ammonium and nitrate produced by microorganisms are converted into these compounds. Animals can then obtain the amino acids they need through consumption of plants, microorganisms, or other animals
- Biological availability. They are readily usable by all organisms.
- Reservoirs and fates. These are bonded together into long chains which are then folded into proteins. The specific form and function of the resulting proteins depend on both the identity and sequence in which they are linked. Note that proteins can be decomposed into inorganic nitrogen-containing compounds within organisms. These products are released into soils and waters through processes such as urination. Another group of microorganisms may convert them to amino acids (assimilation) or begin the process which ends with the re-formation of N2 gas (denitrification).
- Important pathways. Assimilation, decomposition, and excretion influence their distribution.
Nitrogen fixation requires specific conditions
The most common form of nitrogen, N2 gas, is certainly abundant on Earth—we are surrounded by it—but its two atoms are not useable if they remain bonded together. Complicating matters, it is rather difficult to separate them, and only a limited number of organisms can break that triple bond (see the structure, above) and incorporate the resulting N atoms into ammonium and nitrate. The chemical transformations that increase the biological availability of nitrogen are therefore both relatively rare and essential to the biosphere. Here, we will take just a brief look at the process.
Organisms. About 85% of the nitrogen fixation on Earth is carried out by organisms. Lightening and other phenomena can also lead to the conversion of some nitrogen gas to ammonium, but these forces are relatively insignificant. Certain bacteria have the capacity to carry out the first of many steps in nitrogen fixation. Omitting many of the details, different nitrogen-fixing bacteria carry out this or a similar process under varying conditions. Some do it inside of specialized plants, living cooperatively with the host organism. In these cases, the plant receives useable nitrogen compounds, and the bacteria receive a suitable habitat and fixed carbon compounds (see Chapter 5). Other bacteria fix N while living independently in soil or water.
Environment. In addition to the energy requirement suggested in the reaction above, biological nitrogen fixation requires an environment that lacks O2 gas because the enzyme that catalyzes the reaction is poisoned by oxygen.
Movement between bioavailable and non-bioavailable forms is key
As with carbon, both chemical form and location of atoms are relevant to the nitrogen cycle. It is fair to say that environmental scientists pay a great day of attention to those forces affecting biological availability because of how limited fixed nitrogen can be in certain systems. Speaking broadly, then, we can visualize two possible directions or pathways within the nitrogen cycle: one associated with increasing bioavailability and one associated with declining availability (Figure 4.6 provides an overview of the processes and Figure 4.7 provides more details).
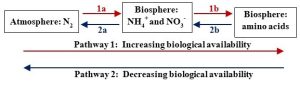
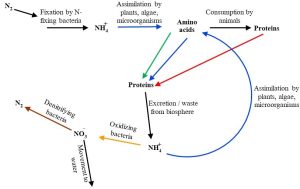
The phosphorus (P) cycle.
Phosphorus is essential for organisms
The availability of phosphorus has a profound influence on organisms. If it is lacking, they cannot effectively store energy, synthesize proteins, encode genetic information, and, in animals, construct bones and teeth. It is also important to us as environmental scientists because of its roles in the stories of ecosystems (Chapter 5), agriculture (Chapter 9), and water pollution (Chapter 11).
Phosphorus is part of many chemical compounds
Both organic and inorganic forms of phosphorus are possible, and many of Earth’s systems depend on P for proper functioning. For the purposes of this discussion, though, we will simplify matters and only consider whether P is present in a particular system. The effect of chemical form, so critical to the carbon and nitrogen cycles, will not be of concern here.
The distribution of phosphorus depends on slow processes
Much of Earth’s phosphorus is stored in the lithosphere and the oceans, and only a miniscule amount is in the atmosphere. Since phosphorus has no relevant gas phase, it moves far more slowly than do carbon and nitrogen. This low rate of recycling is important: because phosphorus is often in short supply relative to demand for it, both natural and human systems are limited by its availability.
Uptake by terrestrial organisms. The word uptake is often used by environmental scientists to refer to the movement of materials into organisms (we will see it in future chapters as well). So, varying mechanisms like ingestion through the mouth of an animal, absorption across the cell membrane of a microorganism (Chapter 3), and even movement into plant roots are typically grouped together.
Excretion and death. As we have seen before, waste products and remains contain important elements. Decomposition releases some of the stored phosphorus, making it available for uptake by a new group of soil dwellers. Some of the phosphorus binds to soil, though, decreasing its availability and increasing the likelihood that it will be lost from the system (see the next item on this list).
Movement with water. Some of the solid materials to which phosphorus has bound can be dislodged by flowing water and carried away. As a result, there is net movement of this important element from land through surface runoff and rivers to the ocean (we will study water movement later in this chapter).
Uptake by aquatic organisms. Certain aquatic microorganisms will absorb phosphorus from water, and consumption will move it into larger members of the biosphere.
Deposition onto land. A portion of the phosphorus in the ocean moves into seabirds that eat fish and other marine organisms. Since these birds tend to nest in the same coastal regions year after year, a large amount of their excrement, known as guano, accumulates in selected areas. Guano is rich in phosphorus and other nutrients, so it is part of an important, albeit quirky, pathway for this element. Countries in the western part of South America, for example Peru, once generated a lot of income by mining guano from shockingly large piles and then exporting it (Figure 4.8). Currently, this valuable resource tends to be used within the countries that have it to support their local agriculture.
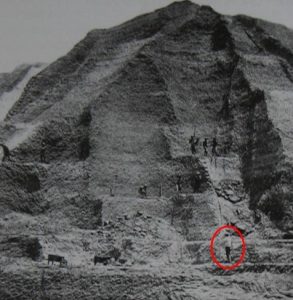
Accumulation on the ocean floor. Phosphorous in the waste and remains of aquatic organisms is generally bound to solids, rather than dissolving in water, and sinks to the bottom of the ocean. Once incorporated into sediments, the nutrient can stay in place for thousands or even millions of years.
Uplift. Although it is nearly impossible to see in the short run, the surface of the Earth is constantly in motion: rocks, sediments, and other materials on the bottom of the ocean can rise up to become dry coastlines and even tall mountains millions of years later (Chapter 3). Given enough time, then, phosphorus on the seafloor will move back to terrestrial areas and be taken up by soil-based organisms again. The processes responsible for uplift are extremely slow, with rates of input of phosphorus into land-based systems lagging far behind rates of output of the element from them.
Upwelling. Some phosphorus in ocean-floor sediments is moved into surface waters due to interactions among wind, Earth’s rotation, and ocean currents that lift deep water (Figure 4.9). Due to the abundance of nutrients in them, coastal areas subject to upwelling are typically teeming with marine life.
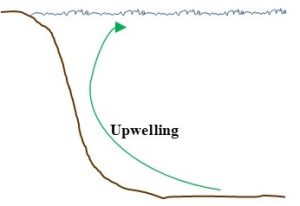
Phosphorus moves along pathways among reservoirs
The phosphorus cycle lacks a gas phase, so, as described above, recycling of this vital nutrient can be very slow. Figure 4.10 is a simplified model of the phosphorus cycle. See its legend for more details.
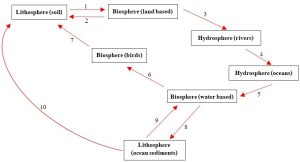
1. Phosphorus in soil is taken up by plants and microorganisms and then moves to animals through consumption.
2. Waste products and remains of organisms return some phosphorus to soil.
3. Some phosphorus is carried away from soil in flowing water. Much of the water ends up in rivers.
4. Rivers carry phosphorus to oceans.
5. Marine microorganisms take up phosphorus from water and pass it to the animals that consume them.
6. Consumption moves some phosphorus from fish and other marine organisms to seabirds.
7. Phosphorus is returned to soil in coastal regions via bird excrement.
8. Much of the phosphorus is not passed to birds but settles to ocean sediments with waste and remains.
9. Upwelling can bring phosphorus into surface waters, making it available for marine organisms.
10. Uplift can slowly push phosphorus in rocks and sediments on the ocean floor up onto land.
Elements must be present in the right amount
Recall the principle described near the beginning of the section on biogeochemical cycling: both too much and too little of a necessary nutrient can be detrimental to systems. Organisms will neither grow nor reproduce effectively if the supply of an element such as phosphorus cannot meet demand. On the other hand, excess phosphorus (in a form called phosphate) is problematic because it can lead to eutrophication, a dramatic and typically undesirable phenomenon affecting aquatic systems. Note that nitrogen and phosphorus can both bring about eutrophication, so we will consider them together here.
Sources of excess nitrogen and phosphorus. Many processes add P and N to aquatic systems. We learned earlier that these two elements can enter water via natural pathways involving organisms, leaching, and runoff. Human activity can release them as well. For example, farmers often apply phosphorus and nitrogen to soil in fertilizers when natural quantities of nutrients are too low to support crop growth (Chapter 9). These and other synthetic chemical compounds not taken up by plants, bound to soil, or otherwise removed, are transported in flowing water to rivers, lakes, ponds, and oceans. Detergents and some industrial products released into waterways can also be an important source of what is sometimes termed nutrient pollution or cultural pollution (note that detergents, a big problem historically, have become a less important P source in recent years due to changes in laws regarding their formulation).
Input-output analysis. Recall what happens when a substance is added to a system faster than it is removed from it: the substance accumulates (Chapter 2). Eutrophication occurs when the rate of input of phosphorus and nitrogen to a small aquatic system (e.g., a pond) exceeds the rate of their output from it. It can also be a problem in larger systems such as rivers and even coastal oceans if very large amounts of nutrients are involved.
Steps in eutrophication. When nutrients accumulate, an important series of events is set in motion that can transform a clear pond into a murky swamp or even dry land. In systems terms, an imbalance in inputs and outputs leads to positive feedback and an ever-increasing rate of change. See Figure 4.11, below, for more information.
- Nitrogen and phosphorus from natural and human sources enter a pond faster than they are removed.
- The excess nutrients stimulate growth of algae and bacteria.
- Algae grow so rapidly and that they form a floating mat on the surface of the pond (Figure 4.12, below). Since little sunlight can penetrate the water, aquatic plants and other carbon-fixing organisms can no longer carry out photosynthesis (C cycle, above). Thus, very little new O2 is added to the pond from within. The mat also restricts movement of O2 from the atmosphere into the water. Oxygen content in the water drops precipitously as aerobic organisms use it up faster than it is replaced.
- When oxygen levels drop low enough, aerobic organisms that dominated the pond prior to the addition of excess nutrients begin to die (such as fish—review Figure 1.4).
- The remains of the dead fish from step 4 are decomposed by certain microorganisms. At first, this process is carried out aerobically. Eventually, though, anaerobic organisms take over when O2 in the water disappears. Some of the products of decomposition, including organic C, N, and P compounds, start to accumulate. The availability of more and more nutrients further stimulates decomposers.
- High rates of die-off of aerobic organisms lead to accumulation of their remains because decomposition cannot keep pace. The water gets increasingly murky, cloudy, impenetrable to sunlight, and inhospitable to all but anaerobic microorganisms.
- The pond gets filled with organic remains and other debris, turning it into a swamp.
- If allowed to continue, the process will eventually dry out the pond, and a terrestrial system will be the result. Land-based organisms like trees, grasses, and animals will dominate the area.
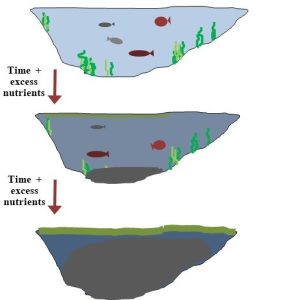
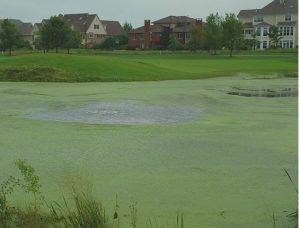
More about feedback and eutrophication. In eutrophication, accumulation of nutrients stimulates the growth of microorganisms and the changes they cause. As less and less oxygen is present in the water, more and more aerobic organisms die; the nutrients in the remains drive more growth of microorganisms and even greater demand for oxygen. Thus, eutrophication is an example of positive feedback: the transition from pond to land accelerates with time (Chapter 2).
4.2.3. Cycling of water
As with other materials, the amount of water on Earth is finite. Since all living and many non-living systems require readily available water to function properly, it must be cycled and recycled rapidly enough to meet demand. The many reservoirs and pathways responsible for the storage and movement of water are linked to make up the hydrologic cycle, one of the most dynamic and important systems we will see (a summary is presented in Figure 4.13, with details to follow).
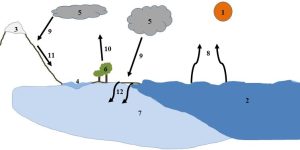
1. The sun, the source of energy for the hydrologic cycle.
2. Oceans, the largest reservoir of water on Earth.
3. Ice, the second largest water reservoir (affected by freezing and thawing, not pictured).
4. River, one example of a freshwater reservoir on the surface.
5. Atmosphere, a reservoir in which water can be found as a gas, liquid, or solid (shown as clouds in this diagram).
6. Trees, one example of a living reservoir of water.
7. Groundwater, a reservoir beneath the surface.
8. Evaporation is a pathway that moves water from the surface to the atmosphere.
9. Condensation followed by precipitation moves water from the atmosphere to the surface.
10. Transpiration moves water from soil to plants to the atmosphere.
11. Runoff, water flowing along the contours of the surface, is one of the fates of precipitation and melt water.
12. Infiltration, water percolating into the ground, is another fate of precipitation and melt water. Kelsey, CC BY-NC-SA.
Water is stored in natural reservoirs
There is a lot of water on this planet, around 326 million trillion gallons of it. However, water is by no means evenly distributed, and importantly to we humans, almost none of it is directly available for drinking.
Several important reservoirs store water. Here we look at the important characteristics of each, keeping in mind that accessibility and usability are crucial concerns. See Figure 4.14 to get a sense of the relative amounts of water in Earth’s major reservoirs (details about the reservoirs are presented below the figure)[2].
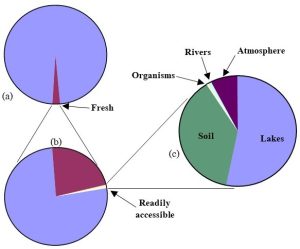
Oceans and seas: 97% of Earth’s water (this and all numbers in this section are rounded; thus, they do not add up to 100%). Nearly all of Earth’s water is stored in salt-water reservoirs. Although the oceans are home to a large and diverse number of organisms, and ocean resources are valued and indispensable, the water itself is not in a form that is consumable by land-based organisms. In other words, almost none of the water on Earth is available for drinking. Sometimes desalination is employed to remove salt and produce fresh water, but that process requires the proper technology, as well as a sizeable financial investment, to carry out on a large scale (more in Chapter 11). Note there is also a small amount of saltwater stored in certain lakes as well as underground (see more about these two types of reservoirs, below), but this combined volume adds a trivial amount to that in the oceans.
Ice: 2%. The water frozen in glaciers and other places on Earth makes up the second largest reservoir. Like that found in oceans, this water is not readily accessible or available.
Groundwater: 0.6% (but 98% of Earth’s fresh, unfrozen water). Since it is not generally as well understood relative to surface waters, and because of its importance as a resource, we will give groundwater some additional attention here.
Rarely seen
This is a reservoir found beneath the surface of the Earth. It can be located as shallow as a meter or less, or as deep as a few hundred meters or more, but in all cases, access to this water is limited by our ability to locate it and pump it to the surface (see Chapter 11 for limitations on groundwater supply).
Rarely in underground pools
Contrary to what is believed by many, the bulk of groundwater is not held in underground lakes and rivers. Yes, some water beneath the surface is stored in open, pool-like structures (notably visible at commercial attractions that allow visitors to explore caverns and the rivers that pass through them), but this is not the typical environment in which groundwater is found. Instead, groundwater tends to occupy the small spaces within rocks, sands, and other porous materials. In a situation that more closely resembles what occurs in a drip coffee maker than that in a channel of a rushing river, water moves downhill, in response to gravity, very slowly through these connected openings (See Figure 4.15).
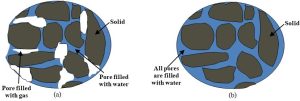
When a layer of comparatively non-porous rocks or clays is present beneath the porous material, water is stopped from further vertical movement. The pores above such an impermeable boundary will then fill from the bottom up. If this accumulation occurs to an extent sufficient to concentrate useable quantities of water, the structure is referred to as an aquifer (more in Box 4.3).
Box 4.3. Next laboratory field trip: the beach!
If you have ever put beach sand into a plastic bucket you have played with a pretty good model of an aquifer. Water you add to the top of the permeable sand will soak down until it encounters the impermeable bottom of the bucket. The top of the sand may appear to be relatively dry, although you know a fair amount of water must be present below the surface because you put it in yourself.
The type of aquifer present in an area depends on several variables, including the arrangement of permeable and impermeable layers. We should make two additional notes about groundwater here. First, not all water under the surface of the Earth is considered part of this reservoir. There is an upper region, known as the unsaturated zone, in which the pores described above contain both water and air. Water here, termed soil water, behaves differently than groundwater and cannot be extracted through wells (soil water is used by soil organisms living in the unsaturated zone). At sufficient depth, in the saturated zone, the air is gone, and pores are filled exclusively with water. Water in this lower zone is classified as groundwater. The boundary between these two zones, which can also be thought of as the top of the saturated zone, is referred to as the water table (Figure 4.16).
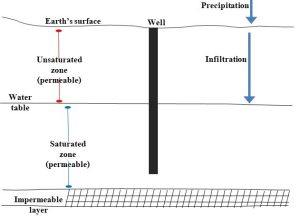
Water flows vertically downward through the unsaturated zone to the saturated zone via infiltration (more below). This movement is critical because it replenishes water that has been removed from the groundwater reservoir (a process known as groundwater recharge; we will see more about the importance of recharge in Chapter 12). Second, in nearly all cases, water must be pumped upward—which can be a great distance—through a well. Very rarely does water rise to the surface naturally. In other words, humans need to invest money and energy if they are to use groundwater. Aquifers are very important to environmental scientists because they often are sources of a substantial amount of water. If they are not managed carefully, demand for water may go unmet. See Chapter 11 for a description of the way human activities can reduce the availability of groundwater.
Fresh lakes, rivers, swamps: 0.01%. These reservoirs are familiar to most people, yet collectively they hold only about 1/100th the amount of freshwater stored below ground. Despite their relatively insignificant volume compared to groundwater, they account for about three times the amount of freshwater withdrawals by humans each day (according to the United States Geological Survey, 230 billion gallons and 76 billion gallons, from lakes and groundwater, respectively[3]). As we will see in Chapter 11, surface water is more susceptible to pollution than is groundwater, so it has its limitations as a resource.
Atmosphere: 0.001%. The bulk of the water in this reservoir is in a gaseous (vapor) form, although there is some liquid here as well. Water in the atmosphere plays many important roles on Earth. We will return to it in the section on precipitation, below, and again in Chapter 14, during our discussion of air pollution.
Soil moisture: 0.001%. A small fraction of underground water is not part of the groundwater reservoir but instead is held much closer to the surface. As we noted above, this reservoir supplies water to plants and other organisms living in the soil (more about soil can be found in Chapter 9).
Organisms: 0.0001%. Individual plants, animals, and microscopic organisms are mostly water, and they cannot survive without a constant supply of it. However, as a percentage of the total amount on Earth, very little water is held in living things.
Water moves along natural pathways
The reservoirs of the hydrologic cycle are neither isolated nor static. Keeping in mind that the net amount of water in each does not change much with time, multiple pathways are responsible for the near-constant movement of water among Earth’s systems[4]. Review Figure 4.13 to see how pathways connect reservoirs.
Evaporation. When heated, water is converted from a liquid to a gas. The sun heats the oceans and other surface waters, so it powers evaporation and ultimately the whole hydrologic cycle. Evaporation accounts for nearly all the movement of water from Earth’s surface to its atmosphere, approximately 90% of it, and all but about 10% of that water returns directly to the ocean through the process known as precipitation (below). The rest ends up falling onto land. The rates of water input and output to and from the surface are roughly equal on a global basis, although there are small-scale systems that experience unequal rates. For example, evaporation generally outpaces precipitation over dry land but occurs at relatively lower rates over bodies of water. In addition to the way it affects the global distribution of water, evaporation can also have a deleterious effect on a small scale (see Box 4.4).
Box 4.4. Dams do not always give you what you expect
Humans often construct reservoirs by diverting or damming rivers. At first glance, this might seem to be a reasonable strategy because water can be artificially stored in areas of high demand rather than being allowed to move downstream. Unfortunately, the transformation of a stream channel into a stagnant, relatively flat lake can dramatically increase the rate of evaporation from an area and provide far less water than hoped (Figure 4.17).
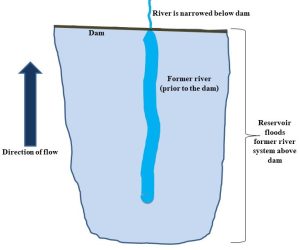
Evaporative loss is the term used to describe what can happen to a substantial fraction of the water trapped behind a dam. Lake Mead, the reservoir created by the Hoover Dam on the Colorado River is just one example of an area affected by this phenomenon. Estimates suggest that approximately 0.75 cubic kilometer (nearly 2 meters of vertical height) of water is lost each year from the lake[5]. Other potential consequences of dam building include decreased flow of water and nutrients to areas downstream, a change in the character of the system that was flooded to create the reservoir, increased risk of flooding if a dam breaks, increased rates of erosion of the stream bed downstream, accumulation of sediments upstream, and interruption of movement of migratory aquatic species such as salmon.
Transpiration. This process is responsible for about 10% of the movement of water from the surface to the atmosphere. In short, water and nutrients enter plant roots, and then nearly all that liquid water moves up and exits as a gas from microscopic openings in leaves. Since it is difficult to separately measure biological and non-biological pathways of evaporation, we often use the term evapotranspiration to refer to all the processes involved in the movement of water from the land to the atmosphere.
Condensation. Condensation is the reverse of evaporation: when water vapor is cooled, it can be converted to a liquid. This phenomenon is responsible for the dew that coats plants and outdoor structures on a cool morning. In the atmosphere, water will condense and combine with very small circulating dust particles to form clouds. At first these wet particles remain aloft, but eventually enough water can be added to them that they become too heavy to stay in the atmosphere. The result is precipitation, the next pathway on our list.
Precipitation. Water that moves toward Earth’s surface due to the force of gravity is known as precipitation. It includes solid forms like ice and snow as well as liquid rain. Precipitation is critical to continuing the hydrologic cycle because it is the principle mechanism by which water returns to the ground from the atmosphere. It supplies organisms with the moisture they need to survive, replenishes the reservoirs described above, and is an important agent in the cycling and recycling of Earth’s crust and rocks (Chapter 3).
Runoff and Infiltration. Rain that hits the ground experiences two important fates: it can flow across the surface, following contours of mountains, hills, and valleys, or it can move downward, penetrating the ground and percolating slowly through soils, rocks, and other materials making up the Earth’s crust. The first of these fates is termed runoff. Water that encounters a material that is not immediately permeable will move from high to low elevation along the surface. For example, water will flow over rocks, hard soil, pavement, buildings and other human structures. This pathway is very important because it replenishes surface reservoirs such as streams and lakes. It also can lead to flooding during rainfall events. Note that for the purposes of this discussion, runoff is classified as a pathway because it is temporary phenomenon that comes after a precipitation event; rivers and streams are grouped with reservoirs because although they clearly move water, they also hold water on a long-term basis. Infiltration is the second fate of water falling to Earth’s surface. Materials such as soil and gravel are permeable, so water will move vertically downward through them in response to gravity. Infiltration is an important pathway because it replenishes water that is removed from underground reservoirs by both human and non-human activities. The balance between infiltration and runoff is of concern to environmental scientists because it has a substantial effect on the likelihood that a rain event will cause flooding along nearby water ways. In areas having relatively impermeable surface materials, runoff will outpace infiltration. Therefore, water will move more efficiently downhill to streams than in areas in which water is diverted as it percolates into and through soils and rocks. We will see more about flooding in Chapter 7.
Freezing and Thawing. Water can be locked up in glaciers or other frozen reservoirs when it freezes. Thawing will release water, subjecting it to evaporation, infiltration, and runoff. Some high-latitude regions on Earth depend on spring thawing to replenish depleted reservoirs.
Consumption and Excretion. As we know, organisms are by far the smallest reservoir of Earth’s water. These pathways are similarly trivial. However, water does enter and exit organisms, and biological usage of water can have an impact on a local scale. Environmental scientists are particularly interested in the ways human activity can pollute and limit the supply of freshwater, a subject we will explore in Chapter 11.
4.2.4. Rock and tectonic cycles
The movement and recycling of the materials making up the lithosphere are also important to a study of environmental science. Please refer to Chapter 3 for a review of those concepts.
4.3. MOVEMENT OF ENERGY
There is a good reason the bulk of this chapter has been devoted to the cycling of materials: their movements, transformations, and recycling are necessary to maintain Earth’s closed systems. We would be remiss, however, if we did not consider the energy that powers the many pathways and processes involved in the constant renewal of nutrients and other resources. Therefore, here we briefly explore the sources and behavior of energy, including how the rules governing it are quite different that those that apply to materials.
4.3.1. What is energy?
Most people have at least a basic understanding that energy is related to one’s ability to accomplish tasks. Casual comments such as “I could not possibly run anymore—I’m out of energy” speak to the importance we assign to it: energy is clearly required for life. Environmental scientists use a similar, if more formal, definition: energy is the capacity to do work. What is work? It can be thought of as the product of an effort to change the status or position of an object. So, when you carry a heavy box upstairs you have used energy to do work—you have moved the box. Two important types of energy are briefly described below.
Potential
In short, this is stored energy; it could provide the capacity to do work, but currently is not used to do anything. If you imagine what it would be like to hold a large boulder in place at the top of a steep slope, you have some idea about the nature of potential energy. As long as you keep your hands on it, the boulder will not move—it is ready to roll, though. Another familiar example of potential energy can be seen when you lift a delicate glass up over your head: the higher you hold it, the more energy is stored (by virtue of its ever-higher position). Chemical compounds can also be viewed as stored energy. In certain circumstance, reactions involving them release energy and propel some important changes, such as the production of a protein from the bonding together of individual amino acids (as in the nitrogen cycle, above).
Kinetic
This is defined as energy in motion. In other words, energy that has been stored for some period of time is now mobilized to do work. The boulder imagined above becomes a manifestation of kinetic energy because, once you let it go, its potential energy drives downhill movement.
4.3.2. Energy inputs to Earth’s systems
A lot of work is carried out on Earth. From the movement of organisms to the evaporation of water to the fixation of nitrogen to the movement of tectonic plates (Chapter 3), demand for energy is high. Moreover, since energy is neither recycled nor reused to do the same work again (unlike materials), new energy must be supplied constantly. Essentially all of Earth’s energy demand is met by three sources.
Solar energy
This is by the most important source of energy to Earth, providing over 99.9% of the energy we receive. It should not be surprising, then, that most systems are powered by the sun, including the hydrologic cycle, all but a small fraction of the biosphere (more in Box 4.5), weather events, wind, and other phenomena in the troposphere. We will see how humans make direct use of the sun for energy in Chapter 10.
The biosphere is mostly powered by the sun—nearly all producers use a form of photosynthesis to fix carbon, and then consumers gain bioavailable forms of carbon they need by living off those producers. However, a few specialized bacteria can produce glucose from carbon dioxide without light. They use energy released in chemical reactions to power their work. These organisms are crucial to supporting larger organisms in dark places like the deep ocean (Chapter 5).
Geothermal energy
It accounts for less than 0.1% of the total, but this still is an important source of energy. Recall from Chapter 3 that Earth began as a completely molten body which has been cooling for about 4.6 billion years. Although the outer crust is relatively cool, much evidence suggests Earth’s interior, namely the core and parts of the mantle, are still very hot. This interior heat slowly moves toward the surface and drives the movement of tectonic plates. Dramatic phenomena such as volcanic eruptions and earthquakes (Chapter 7) are therefore powered by geothermal energy. Humans can tap into this source as well (Chapter 10).
Gravitational energy
This energy is associated with the pull that all objects in space have on each other. The two most important to the Earth are the moon, due to its proximity, and the sun, due to its proximity and size. In simple terms, the changing position of the sun and moon leads to the familiar phenomenon of high and low tides (many other factors beyond the scope of this textbook contribute to the cycling of the tides). Although the amount of energy here is a very small percent of the total—even less than that contributed by geothermal—it still drives a process that affects human and non-human systems along coastlines in profound ways.
4.3.3. Energy outputs from Earth
Energy that enters Earth’s system experiences two fates: it is used to do work and irreversibly changed in the process, or it is reflected back to space, unchanged, without being used to do any work.
Conversions
Energy is converted from forms that have the highest capacity to do work into forms that have the least capacity to do work. Importantly, energy does not disappear from the universe—it is simply transformed. These are such fundamental ideas that scientific laws (Chapter 2) have been developed to describe them. The two most relevant to our discussion are included here.
The first law of thermodynamics. This law states that there is a fixed amount of energy in the universe: energy can be neither created nor destroyed. The energy we saw stored in chemical compounds and then released, for example, is not produced from scratch, it is simply freed or made available to do work. Another way to express the first law is to say that the amount of energy in the universe is conserved.
The second law of thermodynamics. Energy cannot be destroyed, but it is certainly subject to change when it is used to do work. That is, energy undergoes conversions among many different possible types. The second law of thermodynamics describes the nature of these energy conversions. Its essence consists of the following ideas.
Energy is degraded
To understand this concept, we can imagine high-grade energy as concentrated and highly ordered. It becomes less concentrated and less ordered any time it is converted to another form (e.g., after being used to do work). Eventually, the energy is completely dissipated. Keep in mind that all reactions and conversions lead to an increase in the total amount of disorder, known as entropy, in the universe.
Energy loses its ability to do work
Energy conversions occur whenever energy is used to do work. Loss in order, therefore, is linked to lowered ability to do work. Once it becomes dissipated and disordered, energy cannot be used to do the same work again (see Box 4.6 for a familiar example of energy degradation).
Box 4.6. Your car must obey the laws…..of thermodynamics
The next time you are filling up your car’s gas tank, you ought to contemplate the second law of thermodynamics. Consider it takes energy to perform the work of moving your vehicle down the street. If it is like nearly every other car on the road, it has an engine that can convert the potential energy in petroleum into the kinetic energy of moving pistons, spinning wheels, and forward motion. Now, you might ask just how is gasoline used to make the car move? In simple terms, when it is burned, chemical reactions lead to the release of energy. Some of that energy is converted into useful work, like turning of the wheels, but far more than half of it is lost from the system as heat. What happens to the heat? The answer can be found if you touch the outside of the hood of a car that has been running for a few minutes: it is warm (the tailpipe and other components are also warm, but you ought to keep your hands off them). This heat is radiated from the car to space and cannot be used to perform work. As is likely familiar to you, after the car has been driven for several hundred kilometers, it will require more gasoline. At this point your choices are to find a station to refill the tank, that is, provide new high-grade energy and enable additional work, or allow your car to run out of gas. If you want to continue your journey with the second option, you will need to do the work yourself by walking, biking, etc. Be sure you have enough fuel stored in your body before you embark. Once your tank is full and you have paid, you should think about one final, yet crucial, idea. What is the origin of the potential energy stored in the fuel you just purchased? Present-day reservoirs of materials like oil and natural gas are the result of millions of years of processing of the remains of ancient organisms (more in Chapter 10). Recall from our discussion of the carbon cycle earlier in this chapter that some fraction of dead organic matter escapes decomposition and can be transformed into fossil fuels. So, most of our current technology is powered by extremely old organic chemicals never fully broken down by members of the biosphere. It is fair to say your car runs on indirect solar energy!
Earth constantly needs new energy
Once high-grade energy in Earth’s system is degraded, it moves out to space in the form of heat (low-grade energy) and cannot be used again. Only new high-grade energy can replace lost energy if additional work is to be done. In other words, Earth must remain open with respect to energy. In this context, you should review the straight-arrow model used in Figure 4.2 and note how the tail is not connected to the head: again, unlike materials, energy is not recycled.
No energy conversion is 100% efficient
Imagine a hypothetical example involving two types of energy. The first is classed as high grade relative to the second, but both still have the capacity to do some work. Some fraction of the useable energy present in the first form can be converted into the second form. However, much of the energy in the first form never makes it to the second form. Instead, it is completely degraded to heat during the conversion.
Energy on Earth may be dissipated in steps
There are several possible fates of energy. In all cases, high-grade is converted to low-grade energy. The specific number of reservoirs and pathways involved can vary, however. Some of the conversions involve individual small steps from one relatively high-grade form to a lower-grade form, whereas others change the energy from the highest to lowest in one step. The path that energy can follow as it moves from the sun through the biosphere and out to space is modelled in Figure 4.18.
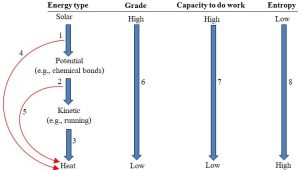
1. Some of the high-grade solar energy is absorbed by photosynthesizing organisms, say, plants. They convert it to chemical bond energy and store it in their cells. Some of that energy is passed to plant-eating animals and stored in their cells.
2. Some energy stored in animals is converted to work: for example, envision a plant-eater chased by a carnivore.
3. After it is used to do the work of running, the energy is converted to heat and radiated off both animals.
4. No energy conversion is 100%; some of the solar energy is lost to heat during the conversion to chemical energy in step 1.
5. The conversion from potential to kinetic energy shown in step 2 is inefficient. Some of the stored energy is converted to heat and not used for running. This inefficiency would mean the bodies of the running animals would feel warm.
6. There is a progressive and irreversible change from high-to low grade energy with each conversion.
7. The capacity for a particular form of energy to do work decreases with each conversion.
8. Entropy increases as energy is used to do work. Kelsey, CC BY-NC-SA.
Reflection
A portion of the sun’s energy striking any planetary body is not absorbed. Just how much is lost this way can be expressed as albedo, the fraction of incoming radiation reflected off a surface. Earth has an albedo of about 0.30, meaning that 30% of light hitting it bounces back to space, whereas Mercury and Venus reflect around 7% and 70%, respectively[6]. Albedo matters because it affects Earth’s temperature; as more energy is absorbed, the warmer the planet becomes. Several factors can change reflection, either increasing or decreasing it. Perhaps most familiar is the tendency of darker colors to absorb more energy than lighter ones. Ice, snow, and other materials will absorb less than will soil, asphalt roads, and most buildings. Green vegetation and water are intermediate in their reflection. Since Earth’s surfaces can change color, the amount of heat absorbed can also change. Consider, for example, what could happen if large-scale transformations of forested land (mostly green leaves) into farms (mostly brown soil) occurred: less energy would be reflected and the temperature of the Earth could increase (Chapter 9). Urbanization would have a similar impact. The melting of ice and snow can also enhance absorption and bring on higher temperatures as more and more dark soil is revealed. We will return to the effect of albedo on temperature in Chapter 14 when we consider human activities influencing Earth’s climate. Figure 4.19 is a simplified representation of the sources, pathways, and fates of energy that enters Earth’s system from the sun.
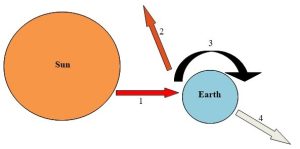
1. High-grade solar radiation strikes the Earth.
2. About 30% of incoming radiation is reflected to space.
3. Energy not reflected is absorbed and used to do work. It undergoes conversions from high- to low-grade forms (see the main text).
4. After it has been degraded, energy is released to space from Earth as heat. Kelsey, CC BY-NC-SA.
THE CHAPTER ESSENCE IN BRIEF [7]
Earth is closed with respect to materials, thus environmental scientists carefully track the movements, transformations, and storage of materials within and among the atmosphere, hydrosphere, biosphere, and lithosphere. Energy behaves differently than materials, so Earth must remain open with respect to it.
Think about it some more…[8]
Are the four spheres of the Earth separate entities or do they overlap?
Is more always better when it comes to essential nutrients? Think about the nitrogen and phosphorus cycles as you ponder your answer.
How are biogeochemical cycles good examples of the fundamental principle that Earth is closed with respect to materials (Chapter 1)?
Why might we care about the rate of evaporation from a constructed water reservoir?
How is most groundwater stored (under natural conditions)?
What does energy enable?
What is wrong with comment such as “we have run out of energy”? Is there a more appropriate way to put it?
- Altitudes in the Atmosphere according to data provided by NASA (NASA.gov). ↵
- Volumes of water according to The US Dept. of The Interior (DOI.gov) and U.S. Geological Survey (usgs.gov) ↵
- The US Dept. of The Interior (DOI.gov) and U.S. Geological Survey (usgs.gov) ↵
- Relative importance of pathways according to USGS (usgs.gov). ↵
- Lake Mead's Water Budget, National Parks Service. 2023. Public domain. ↵
- NASA. Mercury and Venus fact sheets. 2021. Public domain. ↵
- As you will find throughout this book, here is very succinct summary of the major themes and conclusions of Chapter 4 distilled down to a few sentences and fit to be printed on a t-shirt or posted to social media. ↵
- These questions should not be viewed as an exhaustive review of this chapter; rather, they are intended to provide a starting point for you to contemplate and synthesize some important ideas you have learned so far. ↵
The inner-most layer of the atmosphere, this is where weather events occur and is the portion of the atmosphere that is in direct contact with Earth's surface and living things. See Chapters 4 and 14 for more.
Terms referring to something that degrades the quality of air, water, or soil. The term 'pollutant' is used to refer to that material that degrades quality. Compare with 'contaminant', a word with a related, if distinct, meaning. In short, a contaminant can be thought of as any substance that does not belong where it is found (i.e., a metal dissolved in water). A pollutant is a contaminant that has reached a high enough concentration to cause adverse effects (i.e., that metal noted in the previous sentence is at such a high level that it is killing fish; see Chapter 11 for more about water pollution). Note that the terms are often used interchangeably by scientists and members of the public alike.
A geology term referring to the thin, brittle outer layer of the Earth. It is composed of relatively low-density elements. See Chapter 3 for more.
A geology term that refers to the middle zone of the Earth that sits between the crust and core. It is thick, mostly plastic in behavior, and has a higher density than does the crust. See Chapter 3 for more.
Refers to the many different kinds of microscopic organisms that are single celled and prokaryotic (i.e., do not possess distinct cellular organelles). Collectively, bacteria are extremely diverse, are the oldest organisms on Earth, and are critical to many environmental cycles and processes. See Chapter 3 for more.
A group of organisms that are microscopic, eukaryotic, mobile (also known as "motile"), and usually heterotrophic. They are roughly ten times larger than bacteria; they also tend to prey on bacteria. See Chapter 3 for more.
A group of organisms that are eukaryotic, phototrophic, and autotrophic. Some are unicellular, but most are colonial. Note: some scientists include certain prokaryotic cells in this group, the so-called "blue-green algae"; others classify those prokaryotic organisms as bacteria (known as "cyanobacteria"). We will follow the latter convention in this book. See Chapter 3 for more.
Classed as eukaryotic microorganisms. Some are unicellular (e.g., yeast), many are multicellular (e.g., mushroom). They are are heterotrophic and tend to act as important decomposers in ecosystems. See Chapter 3 for more.
An acellular biological entity. Viruses are not alive in the same sense that cellular plants, animals, fungi, bacteria, and protozoa are alive, but they carry genetic information and can reproduce using the machinery of cells in organism they invade. Viruses are extremely small, 100-1000 times smaller than most bacteria and 1000-10,000 times smaller than most eukaryotic cells. They are important agents of disease. See Chapter 3 for more.
A gas made up of one carbon atom and two oxygen atoms, it is one of the many forms carbon can take. This gas is the product of aerobic respiration as well as combustion of fossil fuels, wood, and other materials containing organic carbon. It is not directly usable as a carbon source but can be fixed into useable forms of carbon, for example glucose, by autotrophic organisms. See Chapters 4 and 14 for more.
Formally, energy is the capacity to perform work. It enables changes to be made to matter. For example, energy allows you to move a box from the floor to a table. See Chapter 4 for more.
Positively charged subatomic particle found in the nucleus of an atom. The number of protons varies by, and defines, atom type (i.e., atoms of specific elements are defined by the number of protons in their nuclei). See Chapter 4 for more.
In chemistry, a subatomic particle found in the nucleus of an atom. These are uncharged. Different atoms of the same element may vary in the number of neutrons they possess, even as they must have the same number of protons. Atoms having the same number of protons but different numbers of neutrons are called isotopes. See Chapters 4 and 10 for more.
In chemistry, a negatively charged subatomic particle that orbits an atom's nucleus. Neutral atoms contain the same number of protons and electrons. See Chapter 4 for more.
One of the known elements in the Universe, carbon (C) atoms consist of 6 protons and 6 electrons. Carbon is an important element for living things; organic C compounds make up the skeleton of biological systems. See Chapter 4 for details.
In chemistry, an adjective that refers to chemical compounds that consist of at least one C atom that is bonded to at least one H atom. Compare to inorganic. See Chapter 4 for details.
In chemistry, refers to chemical compounds that lack at least one of the following: a C atom and at one C-H bond. Compare to organic. See Chapter 4 for details.
Refers to natural sites in which important materials such as water, petroleum, nitrogen, and many others, are stored. See Chapter 1 for more.
Refers to natural processes that move materials among earth's natural reservoirs. For example, evaporation moves water from surface reservoirs like the ocean to the atmosphere. See Chapter 1 for more.
A geology term referring to distinct units of the lithosphere. Several of these large plates make up the lithosphere, and they move around, on top of the asthenosphere, with respect to each other. Their movements are responsible for many important phenomena, including continental drift, earthquakes, and volcanoes. See Chapter 3 for more.