15 Human-Health and Environmental Toxicology
Jason Kelsey
Toxicology is a wide-ranging and important science that profoundly affects our daily lives. Toxicologists, the scientists who work in the field, draw upon knowledge from multiple areas, including biology, physiology, chemistry, mathematics, physics, soil science, and ecology to study the effects of poisons on biological systems.
Before proceeding any further into this final chapter, we will first take a moment to reflect on some of the ideas we have considered thus far. Clearly a few sentences cannot fully summarize 14 chapters of a textbook, but some common themes and trends stand out. First, living and non-living systems are inextricably bound together: as we know well, they influence each other in multiple, complex ways. Second, anthropogenic activity, particularly that of the industrialized era, has profoundly affected Earth’s environments. Third, people depend on natural processes for survival as well as health, prosperity, happiness, and other hard-to-quantify benefits.
Why the sudden walk down memory lane? Well, the science of toxicology touches on and grows from many of those ideas we have studied already. For instance, as humans seek to fulfill our expanding demands for living space, long life, transportation, sustenance, and power we introduce many foreign substances, some of which are harmful, into our bodies, as well as to soil, air, and water. Put another way, the chemicals that are so integral to our modern lives also have the potential to adversely affect the health of both human and natural ecological systems.
Key concepts
After reading Chapter 15, you should understand the following:
- The long history and continuing relevance of the science of toxicology
- The fundamental principles of toxicology, including dose-response and selective toxicity
- How toxicological data are collected and expressed
- How poisons enter and move within organisms, are metabolized, and are excreted
- The multiple potential consequences of exposure to poisons
- The principles of environmental toxicology, including how toxic substances can affect ecosystems
- The fundamental principles of risk assessment and how they can be used to improve human safety
Chapter contents
15.1. Toxicology Has Ancient Roots
15.2. Sources of Poisons
15.3. Fundamentals of Modern Toxicology
15.4. Consequences of Exposure: a Deeper Dive
15.5. Environmental Toxicology
15.6. Risk Assessment
The Chapter Essence in Brief
15.1. TOXICOLOGY HAS ANCIENT ROOTS
Humans surely have been interested in poisons for as long as they have understood cause and effect. The first people to connect a red, itchy skin rash with rubbing up against something like poison ivy or stomach cramps with ingesting wild berries were, arguably, the earliest toxicologists. Later and more formally, the ancient Egyptians, Greeks, and Romans methodically studied and then employed poisons in warfare, assassinations, and executions. Others, such as early indigenous North Americans, harnessed the power of natural substances like peyote not to cause harm, exactly, but to alter their mental state and consciousness. In these and other cases, people first learned how a wide range of substances can affect humans and then proceeded to make use of their accumulated knowledge. A full accounting of the history of toxicology is neither necessary nor practicable here. Suffice it to say, toxicology is among the oldest human sciences. See Box 15.1 for one quirky historical example of inspired toxicology gone spectacularly wrong.
Box 15.1. Warrior, king…toxicologist?
We take a moment now to consider the fascinating and grisly tale of a somewhat obscure historical figure known as Mithridates VI. This remarkable man was the leader of ancient Pontus, a country located on the Black Sea in the region of modern-day Turkey. He died during the last century B.C., but not before dramatically adding to our knowledge of the science of poisons as well as making quite a bit of trouble for the Romans (getting right to the point, so much so that they very much wanted to parade him through the streets of Rome before subjecting him to a horrible death). Mithridates, like many of the rulers that came before and after him, feared he would be assassinated by poisoning (his father seems to have died by that fate—at the hands of his mother). To thwart would-be usurpers, Mithridates developed a concoction of small amounts of common poisons that he ingested prophylactically for years. His intentional low-dose exposure effectively ramped up his natural defenses and resistance to a range of toxic substances, allowing him to survive, rule his people, and annoy Rome for decades. It really was a brilliant feat, one that influences toxicologists and physicians to this day. However, a downside to this act of inspired genius eventually reared its head. If we fast forward to his final hours, we find the mighty scientist under siege and trapped by a Roman army ready and excited to finally take him prisoner. Knowing the fate awaiting him upon capture, Mithridates decided to kill himself before it was too late.
The end of this story nearly writes itself: the superhuman resistance that worked so fantastically well for so long also protected him against any of the available poisons that could have killed him gently when he actually wanted to die. Sadly, he had to opt for the far more painful method of falling on his servant’s sword to get the job done.
15.2. SOURCES OF POISONS
Hyperbolic as it may sound, poisons are all around us. We can encounter toxic substances whenever we inhale, eat, drink, or touch anything. Anthropogenic and natural processes, products, and activities, many of which we saw in the previous chapters of this book, are important sources of potentially harmful substances. Here we examine some noteworthy examples. First, however, a word of caution is in order: the source of a substance is no guarantee of safety or risk of it. Many people assume that “synthetic” and “natural” are synonyms for “dangerous” and “safe”, respectively. The world is far too complex for such simple conclusions, as some artificially generated substances are life saving whereas, as we will see below, some natural substances are extraordinarily harmful to humans.
15.2.1. Anthropogenic
Agriculture
Recall from Chapter 9 that fertilizers and pesticides are used extensively in conventional agriculture. Yes, such chemicals generally enhance food production, but they also can harm living systems.
Fertilizers. Farmers often add nitrogen, phosphorous, and other nutrients to soil. In addition to stimulating crop growth, their movement away from farms can initiate eutrophication and pollute drinking water. Fertilizers provide an important and generalizable lesson, namely, the same chemical compounds that are so helpful in one context can be deadly in another.
Pesticides. This broad group of substances includes many specific categories, two of which are used widely in agriculture: insecticides (they target fruit flies, locusts, and other insects) and herbicides (weeds). Others, including rodenticides (mice, rats, and the like), fungicides (mold), and molluskicides (snails and slugs), are also used to varying extents. Pesticides can be very effective in killing organisms that reduce crop yields, but they also can adversely affect non-target organisms, that is, those we want to protect form harm. For example, a compound deadly to corn-eating caterpillars may also damage pollinating bees, lady beetles, birds, fish, and humans.
Food preparation, packaging, and consumption
The many steps used to transport, modify, prepare, and sell farm products can introduce a variety of biological, chemical, and physical poisons into food. Some, like preservatives, colors, and flavors are intentionally added in attempts to enhance food in one or more ways; these are all classified as additives. On the other hand, metals, cleaning agents, microbial toxins, and so forth that end up in food inadvertently are contaminants. What about the pesticides we noted above? One could argue they have attributes of both additives and contaminants in that we knowingly add them to food crops, but we do not intend for humans to ingest them. However you wish to categorize them, detectable pesticide residues can and do end up on fruits and vegetables, although they tend to be present in small quantities and so pose a minimal risk (as noted in Chapter 9, farm workers exposed on the job are far more likely to experience pesticide-induced health effects than are consumers—see Figure 15.1 as well). The importance of the amount of exposure to, or more correctly, dose, of a poison, is a central idea in toxicology that we will explore in depth shortly.
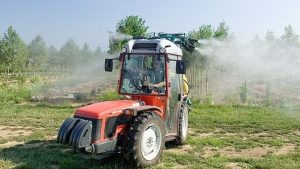
Drugs
This group includes the enormous number of natural and synthetic substances used by humans for therapeutic (i.e., to remedy a debilitating condition or illness) or recreational (i.e., they play no formal medicinal role but provide pleasure of some kind) purposes. Since a full exploration of the story of drugs would entail a great deal of time and space and divert us from our primary path, we will need to settle for a few brief comments on the topic. First, with very rare exceptions, people who take drugs do so to derive some benefit. For example, you might swallow one kind of pill to relieve a toothache and another to combat a bacterial infection in your ear, administer a few drops of liquid into your eyes to reduce redness or apply a cream to help heal damaged skin, inhale a mist to mitigate the symptoms of respiratory allergies, or inject insulin to treat diabetes. Of course, some people swallow, inhale, or inject amphetamines, cannabis, or heroin, respectively, to gratify other desires. Second, drugs like those just listed have primary, intended effects, but they also have the capacity to trigger side effects, outcomes that are at best inconvenient and at worst debilitating or even lethal. A toxicologist would point out that we intentionally expose ourselves to toxic substances with any drug we take, therefore, the risk of potential adverse reactions must be weighed against the potential benefits (review the concept of cost-benefit analyses in Chapter 1).
Cosmetics
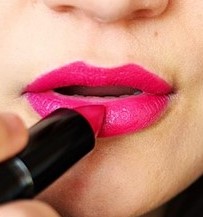
Cosmetics are commonly used substances intentionally applied to exterior portions of human bodies. Like drugs, these products carry risks—they can contain toxic substances that are readily absorbed through skin (things like metals and solvents)—and benefits, hard-to-objectively-quantify changes to one’s appearance, odor, and so forth (Figure 15.2 shows a familiar example).
Power generation and usage
Many of the strategies we use to meet our demand for power, most notably the burning of fossil fuels and harnessing of nuclear fission, can release poisons into our air, water, and soil. The smog tied to coal combustion, for example, contains chemical and physical substances that damage human and environmental health. Radiation from nuclear power plants, although generally contained, also has the potential to cause widespread and severe adverse effects. Review Chapter 10 and Chapter 14 for more about the risks associated with power generation.
Waste production and management
Industrial, agricultural, military, medical, academic, and domestic activity all generate waste products. Steps are taken to minimize pollution by these materials (for example, sewage treatment facilities and landfills). Still, human and natural systems are exposed to chemical, biological, and physical poisons from accidental releases and improper handling of waste. Review Chapter 13 for more about waste management.
15.2.2. Natural
As we noted in Chapter 7, modern humans are not alone in their capacity to create hazards and threaten the well-being of living things. Volcanic eruptions (including the emission of toxic gases), earthquakes, mass wasting, flooding, and diseases are among the natural phenomena and forces that can devastate human and natural systems.
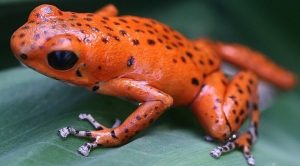
Our list can quickly grow if we add poison-producing animals (think rattlesnakes, black widow spiders, Komodo dragons, and tree frogs, Figure 15.3), heavy metals like arsenic and mercury in rocks, soils, and waters, radon gas, and ultra-violet radiation (UV, see Chapter 14) striking us from the sun. Finally, we should give credit to toxins produced by microorganisms. Consider just one of them, botulinum toxin. This exquisitely poisonous substance is produced by a naturally occurring bacterial species and is more potent than any other known substance. It ought to be clear that Earth offered plenty of risks before we appeared on the scene and continues to do so.
15.3. FUNDAMENTALS OF MODERN TOXICOLOGY
Of course toxicologists are bound by the scientific method that we have seen throughout this book. Additionally, though, they are informed by several concepts that are unique to their science. Here we briefly explore the terms, assumptions, and principles needed to understand toxicology.
15.3.1. Speaking like a toxicologist: essential terms
Xenobiotic (‘zeno bi ä dik’)
Toxicologists frequently use this term to describe any substance that enters an organism from an external source. That is, xenobiotics do not originate within the body of an affected organism. Keep in mind that xenobiotics can bring about a range of reactions, from benefit to harm (some of these we will see shortly).
Poison
This word refers to a substance that causes harm to a biological entity. Poison, and another word, toxin, are commonly used interchangeably, though most toxicologists recognize subtle differences in their meaning. The latter, toxin, is more specific, including compounds that are produced by organisms (i.e., animals, plants, or microorganisms), and the former includes a broader range of substances from any source (i.e., they are natural or synthetic) that are harmful. Importantly, both terms suggest substances that induce adverse consequences in exposed organisms.
Dose
The concentration of a xenobiotic within an organism is known as the dose. It typically is expressed as the amount of the substance in an organism divided by the mass of the organism, i.e.,
amount of xenobiotic (mg) / mass of organism (kg).
Body burden may be used to refer to the total amount of a xenobiotic in an organism.
Response
Response is broadly defined as the consequence(s) caused by a xenobiotic. We also use the term endpoint to indicate a reaction to exposure. Keep in mind that a xenobiotic can initiate a harmful or beneficial response (in fact, as we will see in 15.3.2., the same compound may cause both detrimental and helpful outcomes). Among the many possible endpoints are paralysis, reproductive failure, skin irritation, loss of bone mass, blindness, and death (some details about response are presented in section 15.4). One more caveat is appropriate before we proceed: you should re-read the first sentence in this paragraph and reflect on the word “caused”. Toxicologists establish a mechanistic link between a xenobiotic and whatever response is observed (review the difference between correlation and causation in Chapter 2).
Potency
The dose required to bring about a response is a function of the potency of a substance. This property ranges enormously among xenobiotics. Certain forms of mercury, for example, have high potency in that low concentrations in the body can cause measurable and serious adverse effects. On the other hand, many vitamins and synthetic drugs have low potency, meaning very high doses are required to cause damage. Importantly, accidental overdoses from low-potency compounds are unlikely (although not impossible, as we will see).
Routes of exposure
Exposure refers generally to the critical event during which a person comes into contact with a xenobiotic. Why is exposure deemed “critical”? Because even the most potent toxin known poses no threat unless it is encountered. But we take things a step further than a simple question of whether there was exposure because the specific way a xenobiotic gets into an organism influences how much of and how quickly a potentially harmful substance moves to sensitive tissues like the brain, heart, liver, and others. As suggested in the earlier paragraph about drugs, there are four distinct routes by which a xenobiotic can move into an organism: via gastrointestinal ingestion (GI, you swallow it), dermal contact (through your skin), inhalation (you breathe it in), and intravenously (direct injection into your bloodstream). You should realize that none of the routes is necessarily more or less dangerous than the others. Whether dermal exposure, for example, poses less of a threat than does GI exposure depends on the specific properties of the xenobiotic in question. You will see a related term throughout this chapter, uptake, which is often used nonspecifically to refer to the entry of a xenobiotic into an organism. We will learn more about the relevance of, as well as the factors affecting, exposure shortly.
Target system
A drug or other potential poison has an impact on an organism not because it interacts with all systems at once, rather, xenobiotics tend to preferentially affect one (or possibly a few) sites within the body that toxicologists refer to as targets. Damage at targets then can lead to a variety of consequences (or responses, as noted above) ranging from mild to severe. There are many potential targets within the human body, including the central nervous system (CNS, i.e., the brain and nerves), the respiratory system (lungs and associated structure), the digestive system (stomach, intestines), the cardiovascular system (heart and blood distribution) eyes, ears, skin, bones, and many others. Certain forms of mercury, for instance, cause damage by affecting their target, the CNS. As a result of disruptions to the nervous system, a body may suffer from seizures, paralysis, and potentially, death. Excess fluoride, on the other hand, may cause more localized damage to teeth only (you read that right: we will see more about fluoride below).
15.3.2. Two underlying principles
Dose determines response
We begin this section with the principle of dose-response, an idea first described in the 16th Century by a Swiss scientist named Philippus Aureolus Theophrastus Bombastus von Hohenheim (thankfully, known as Paracelsus to his friends as well as we modern toxicologists). Put simply, Paracelsus noted how the effects caused by a xenobiotic depend on the amount and concentration of that xenobiotic in a body. The story quickly gets nuanced from here because response tends to run the gamut through increasing benefit to increasing harm as dose goes up. For example, a pain killer like acetaminophen will provide no relief if it is taken at a very low dose but will dull your headache once the concentration of it in your blood reaches the appropriate therapeutic level (i.e., after you ingest enough of it). At higher and higher doses of that same drug, though, harm is done to multiple systems until, at the lethal dose, death from liver damage occurs. The same phenomenon is seen with other over-the-counter and prescription medications, even as the specific therapeutic and toxic doses will vary considerably (in case it does not go without saying: you should always read and follow the dosing instructions for any medication!). See Figure 15.4 for a graphical representation of the dose-response principle.
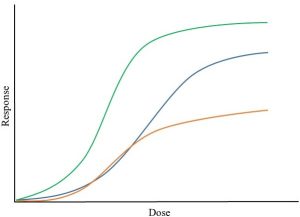
Now, things get even more complicated than we saw in Figure 15.4 because in many cases adverse response increases at both very low and very high doses. How can this be? Consider the relationship between dose and response for an essential nutrient like selenium. A deficiency of this metal—doses approaching 0 mg / kg—is harmful, meaning that likelihood of damage to the organism goes up as dose goes down. However, as selenium levels continue to increase beyond the therapeutic level adverse responses are seen again (see Figure 15.5 for the kind of dose-response curve we would see here).
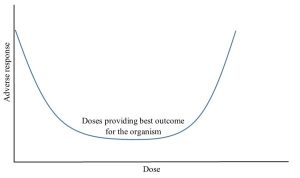
Similar U-shaped curves characterize the dose-response behavior of fluoride (too little and too much can lead to tooth decay) and ethanol (low doses may provide protection against heart disease whereas high doses increase risk from cancer and heart disease). In these and many other cases, there is a range of doses that is best, neither too high nor too low. This phenomenon, known as hormesis, is somewhat controversial within the scientific community for a number of reasons, including the fact that it is difficult to see how it could apply to everything. For instance, lead, mercury, and gamma radiation appear to be harmful at all doses; there is no range within which they provide benefit. Research in this area is ongoing.
Another wrinkle in the dose-response relationship merits a brief mention. We assume that relevant responses occur at all doses greater than 0 mg / kg, that is, even extremely low doses cause responses. Sometimes, however, responses are not evident until a dose appreciably above 0 is reached. Put another way, some minimum dose, one higher than 0, is required before any response is seen. Such xenobiotics are said to display a threshold, formally defined as the dose below which no response is observable (Figure 15.6).
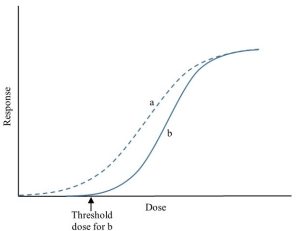
The notion of a threshold may seem reasonable enough, but like hormesis, not all toxicologists accept it. Several objections have been raised, including a question that is embedded in the basic definition of the phenomenon: the dose at which no response is observable. What if the response is too faint to be detected with existing scientific instruments (i.e., we lack sufficient sensitivity to measure it)? In such a case, there would be no real threshold dose, just a difficult-to-see response to a low dose.
How do we determine the relationship between dose and response? The answer requires a great deal of money, work, scientific experimentation, and time (a decade or more is not out of the question). Every new drug (and cosmetic and food additive, for that matter) undergoes a series of tests before it can be sold legally to the public. These tests are conducted hierarchically, meaning we start with relatively simple analyses, including whether the test compound adversely affects cells in petri dishes, and then move on to additional, more complex tests, if initial data warrant such a continuation. Typically, a candidate that appears promising after early stages will next undergo testing in laboratory animals like insects, rats, and mice. In these latter and more complicated tests, groups of organisms from the same species are each given different doses of the xenobiotic and the number of subjects affected is recorded (tests on larger animals, and ultimately humans, will ultimately follow if and when the compound is deemed safe enough for such a step). From these data, the relationship between dose and response within whole populations can be predicted.
Finally, we address one additional, possibly surprising, implication of the dose-response principle. Not only does dose determine whether a substance will serve as a therapy or poison (e.g., acetaminophen and selenium, as we noted earlier), every xenobiotic will be deadly at some dose. Potency clearly plays an important role in whether a compound is more likely to help or hurt, but even the seemingly friendliest and most familiar substances have the capacity to harm. Consider water. We all know it is fundamental to survival, but few realize it can kill if it reaches a high enough concentration in the body (yes, water is poisonous in the range of many liters consumed within a short time period). Toxicologists sum it up this way: the dose makes the poison.
Responses vary among species
Known as the principle of selective toxicity, this second concept is critical to an understanding and application of the science of toxicology.
Explanations and examples. Put simply, different species receiving the same dose of a particular poison will not respond in the same way. Acetaminophen, a compound we have discussed already, provides a vivid example of this phenomenon. Due to some differences in their biochemical properties, humans can tolerate much higher doses of this common drug than can certain house pets; in fact, a very low dose of acetaminophen is likely to kill dogs and cats. Many other xenobiotics and organisms demonstrate the principle, including herbicides (far more lethal to plants than animals), antibiotics (kill bacteria, not animals), preservatives (inhibit microorganisms that can spoil food, but, in principle, do not affect human consumers). In some cases, the reasons for selective toxicity are straight forward: an herbicide targets a cell component that is absent in animals, for example. At times, though, differences in response are surprising and harder to explain, say how a notorious drug like thalidomide causes birth defects in humans but not mice. We will learn more about thalidomide shortly.
Applications. The fact that humans respond differently to certain poisons than do other organisms comes in handy. Many of the substances we encountered above are useful because of their selective toxicity. Think about a drug like penicillin. We take it to treat some illnesses because it kills the responsible bacteria without appreciably affecting the health of most people (review Box 5.6 for more about antibiotics). Why is penicillin selectively toxic? Because it targets the construction of cell walls, structures that humans (and all animals) lack. To varying extents, weed killers, insecticides, fungicides, preservatives, and many others cause less harm to humans than they do to their target organisms. Of course, despite the benefits we derive from selective toxicity, it also complicates the drug-development process described earlier in this chapter because the responses of test organisms may or may not be similar to responses in humans. We will return to this problem in section 15.6.
It also is seen within a species. We would be remiss if we left out this final point about selective toxicity. In addition to differences between species (e.g., humans vs. cats vs. mice vs. bacteria vs. plants…), members of the same species also tend to respond differently to xenobiotics. Genetic variation among humans, for instance, leads to differences in storage, metabolism, and excretion (three protective strategies we will explore in the next section), in other words, intraspecies selective toxicity. Now you may better appreciate the presence of disclaimers that sound something like “individual results may vary” in drug advertisements.
15.4. CONSEQUENCES OF EXPOSURE: A DEEPER DIVE
15.4.1. Xenobiotics may harm organisms
As we noted above, a poison can disrupt one or more sensitive targets. Changes to affected systems may then lead to local and / or whole-organism consequences. We have already listed some examples of target systems and endpoints, but here we will take a closer look at some of particular concern to toxicologists.
Mutations and cancer
As we saw back in Chapter 6, changes to DNA can alter the physical traits of an organism. Mutations also can lead to the induction of abnormal cell growth and the development of rapidly expanding masses known as a tumors, that, left unchecked and untreated, will cause the death of the affected organism. Among the many causes of mutation is exposure to xenobiotics that specifically target DNA. The list of potential carcinogens, cancer-causing agents, is lengthy and includes components of cigarette smoke, certain pesticides, food contaminants and additives, asbestos, industrial products, and many others.
Birth defects
Prenatal exposure to xenobiotics may disrupt the growth of an offspring, despite the extra layers of protection provided by the placenta. Teratogenic compounds (those with the capacity to induce birth defects) can affect one or more crucial stages of development and bring on abnormalities ranging from minor to catastrophic. One of the most important historical teratogens was a drug called thalidomide. Used to combat morning sickness in the 1950s and 1960s in several countries, it killed high numbers of cells in a fetus and led to several thousand babies born with missing or severely shortened limbs (it was quickly taken off the market in the early 1960s).
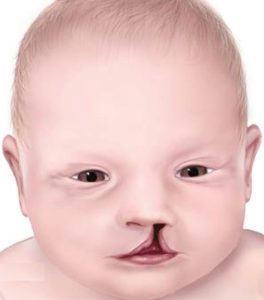
Ethyl alcohol is a second noteworthy teratogen. It has been linked to teratogenesis for centuries and continues to pose a risk if consumed during pregnancy. Unlike thalidomide, it tends to induce subtle, cognitive deficits, although it may cause abnormal physical features as well. Cleft palate, for example, has been linked to alcohol consumption by pregnant women (Figure 15.7).
Nerve damage and paralysis
Several xenobiotics affect the nervous system. Certain pesticides, for example a widely used compound known as malathion, are used because of the way they paralyze target insects. Humans exposed to such compounds can also experience similar life-threatening consequences.
Immune system disruptions
Our immune system is a complex assemblage of cells and tissues that helps protect us from the enormous variety of potentially deadly agents (e.g., bacteria, viruses, venoms, and other toxins) that enter us from outside sources. Damage to it can reduce its effectiveness, an endpoint known as immunosuppression, or ramp up its activity to unhealthy levels: both hypersensitivity (unnecessary reactions to non-threatening agents, essentially allergy) and autoimmunity (inappropriate destruction of one’s own cells) are possible[1]. As with the other endpoints, many xenobiotics cause immunotoxicity. For example, members of the class of compounds known as per- and polyfluoroalky substances (PFAS) found in clothing, cookware, carpets, and industrial chemicals, have been linked to immunosuppression (as well as cancer, birth defects, and liver problems)[2]. Polychlorinated biphenyls (PCBs), another important class of industrial compounds, also act as immunotoxins[3].
Endocrine disruption
The endocrine system uses hormones to control key functions such as reproduction, growth, and metabolism. Certain xenobiotics can induce a range of adverse consequences, including the development of unwanted masculine traits in females (and vice versa), infertility, stunted growth, and others. The list of potential endocrine-disrupting chemicals is long and diverse, and includes the PFASs and PCBs we just noted as well as some plasticizers (e.g., BPA and others found in food storage containers and toys), and naturally occurring phytoestrogens (i.e., estrogen-like chemicals produced by plants such as soybeans)[4].
Organ damage
Our final, broad category includes what its name suggests: disruptions to the normal function of the liver, kidneys, respiratory system, skin, cardiovascular and other critical organs / systems in the body. Many metals, solvents, cleaners, fertilizers, and others have the potential to adversely affect one or more organs[5].
15.4.2. The body can protect itself from harm
At this point you might be wondering: do I have any defenses against xenobiotics, or am I done for? In brief, our bodies have developed many strategies to minimize (if not eliminate) the damage done by xenobiotics.
Barriers to uptake
Remember that a poison can do no harm until and unless it enters an organism. It is likely unsurprising, then, that we possess barriers that reduce uptake of xenobiotics into our bodies and critical body systems. Here are a few of the major protective structures that serve us.
Membranes. Each of the trillions of cells that make up our bodies is enclosed by a cell membrane. Due to their physical and chemical properties, these essential barriers can control much of what goes in and out of cells, including nutrients, waste products and xenobiotics. Keeping things general, only chemical compounds with certain properties can easily cross membranes.
Extra protection for important systems. All internal systems are shielded by cell membranes as noted above, but some of the most critical and sensitive contain extra layers of protection. For example, because of the importance of the brain to overall health and survival, as well as its sensitivity to disruption, penetration of xenobiotics into it is further impeded by several features collectively known as the blood brain barrier. A developing fetus is similarly susceptible to toxic substances so is protected by the placental barrier. Neither system is fool proof and xenobiotics certainty do breach them, but the additional restrictions to penetration reduce risk from life-threatening damage.
Skin. This outer portion of our bodies consists of many layers of cells (with their membranes, of course) and serves as a first line of defense against dermal absorption (recall routes of exposure, above). Skin can inhibit the uptake of some xenobiotics, but many important poisons do cross this barrier and rapidly enter the blood stream.
Storage sites
Recalling the term “target”, we know that systems in our bodies are not equally vulnerable to the effects of a particular xenobiotic. In fact, some tissues are insensitive enough that they can bond to certain compounds without meaningful adverse consequences. Storage sites such as bones, fats, and proteins carried in blood play important roles in binding and therefore reducing the concentration (i.e., the dose) of poisons at target sites. Too good to be true? Alas, yes, a few caveats are in order. First, not all xenobiotics can be effectively stored. Second, less than 100% of a xenobiotic is immobilized, meaning some still circulates throughout the body. Third and critically, storage is not a permanent condition: various processes can lead to the freeing of sequestered poisons and harm to an affected organism.
Metabolism
Xenobiotics that are not stored may undergo chemical transformations by natural metabolic processes within an organism (several body sites participate, although the bulk of this type of metabolism occurs in the liver). Some of these reactions appreciably reduce the danger posed by a xenobiotic, like the ones famously responsible for changing ethyl alcohol into a less-harmful product (thereby reducing the intoxicating effects of wine, beer, and spirits). On the other hand, other reactions actually increase the toxicity of a xenobiotic! What’s with this counter-intuitive maneuver? Well, some of the compounds entering an organism do so in forms that are rather difficult to excrete (see the next defense mechanism on this list). Since eliminating toxic substances is often the best protective strategy, the body goes through a lot of effort to change the chemistry of a xenobiotic such that it is easily dissolved in water and flushed out. Unfortunately, the products of these reactions may be more toxic than the parent xenobiotic (the original compound that entered the body) and can damage some important targets before they exit. In short, metabolism can increase or decrease the likelihood that a xenobiotic will cause harm.
Elimination
The products of metabolism noted immediately above can be released from the body through one of several processes. In terms of the quantity removed, excretion via urination and defecation are the two most important elimination pathways. Other mechanisms of elimination include sweating, movement into hair, vomiting, exhalation, and lactation. Although none of these minor pathways has an appreciable effect on the total amount of a xenobiotic in an adult body, they are not irrelevant; the concentration of poisons in breast milk may be high enough to affect a nursing infant, for example.
15.5. ENVIRONMENTAL TOXICOLOGY
Now we turn our attention to the important branch of toxicology concerned with the sources, fates, effects, and management of pollutants (i.e., poisons) in ecosystems.
15.5.1. Sources of pollutants
As we saw earlier in this chapter, many anthropogenic activities generate and release poisons into soil, air, and water. Fertilizers, pesticides, sewage, drugs, cleaning products, trash, metals, solvents, and many other physical and chemical pollutants can end up in natural systems. Both the number and variety of potentially dangerous substances environmental toxicologists address are enormous.
15.5.2. Fates of pollutants
Whatever its source, a toxic substance can experience one or more fates. That is, pollutants rarely stay put, unchanged, after they are released, instead, they undergo transformations and displacements (i.e., they are moved) that are critical to the health of organisms and ecosystems.
Degradation
This first fate is somewhat analogous to the metabolism of poisons seen in section 15.4, above: some pollutants may be chemically altered by the actions of microorganisms like bacteria and fungi or by non-living phenomena. Often, such reactions transform, or degrade, a relatively large and complex parent compound (using “parent” like we did in section 15.4) into smaller, simple products. Biological processes, or biodegradation, are particularly important in soils because they may reduce or even eliminate the threat from a pollutant. Whether reactions will serve to clean up problematic contaminants depends on properties such as temperature, light and oxygen availability, and water content, though. In other words, if environmental conditions are not conducive to the success of, say, the bacteria that can carry out the necessary reactions, an otherwise degradable pollutant might persist in its parent form indefinitely (see Box 10.2 for a discussion of similar problems in polluted ocean waters). Furthermore, biodegradation does not affect all synthetic chemical compounds, as some are simply inherently difficult for organisms to break apart, nor are all microorganisms able to metabolize relevant poisons. Keeping its limitations in mind, degradation can diminish the likelihood that a pollutant will experience any of the remaining fates on this list. Before addressing them, though, we should revisit another point from the earlier section on metabolism, namely, that chemical changes often decrease the threat from a pollutant, but an increase in toxicity is also possible.
Movement to the atmosphere
In some cases, liquid environmental pollutants change into gaseous forms. This process, known as volatilization, can move highly toxic substances out of soil and into air. Whether pollutants travel to the atmosphere is a function of chemical properties (i.e., some are more likely to volatilize than others) and environmental conditions (e.g., higher temperature increases volatilization).
Movement to reservoirs of the hydrologic cycle
We know that water moves across Earth’s surface via runoff and downward to groundwater via infiltration. Those pollutants that can be dissolved in water will travel out of soil in those same directions, potentially contaminating important reservoirs of the hydrologic cycle (review Chapter 11, including the discussion of leaching). As you probably expect to hear by now, a number of factors influence the extent to which poisons flow with water. First, as noted, only those that are soluble in water are likely to move very far. Second, degradation and volatilization could reduce the amount of pollutant in soil and therefore the amount in water.
Uptake by organisms
Toxicologists use three terms to describe uptake, the entry of environmental pollutants into the biosphere.
Bioconcentration. In this case, a compound moves from non-living soil, water, or air into the cells or tissues of an organism via non-dietary means (i.e., not due to feeding). For example, an earthworm in soil or a fish in water may absorb pollutants through their skin. If you are in a smoke-filled room, you similarly bioconcentrate toxins through your lungs. As usual, the extent to which this process occurs depends on some variables.
Properties of the pollutant
First, pollutants with very low water solubility, for example the insecticide DDT and the industrial compounds PCBs, readily cross cell membranes and then get stored in the fats of organisms. Second, pollutants that are inherently stable—they resist degradation—are more likely to persist long enough to enter organisms (and stay there) than are those with less resistance; DDT and PCBs meet this criterion as well.
Properties of the organism
Although membranes are similar across the biosphere, differences in physiology (i.e., structure of an organism), behavior, and habitat will influence how much of a pollutant will enter an organism. For example, you might imagine that plants, with their root systems, interact differently with soil and the contaminants in it than, say, earthworms, moles, or fungi.
Biomagnification. This second term refers to the movement of a pollutant into an organism by way of diet—that is, it enters an organism through what it eats. So, a small fish can ingest a poison if it eats contaminated algae. That poison will move into a larger fish that eats that smaller algae-eating fish, and so forth up to higher and higher trophic levels. We also describe the movement of a pollutant from prey to predator as trophic transfer.
The factors affecting bioconcentration are similar to those affecting biomagnification. Chemistry of the poison is particularly important because only a compound that persists inside a prey organism is likely to be present by the time a predator arrives on the scene. Furthermore, a compound that does not dissolve in water will move preferentially into fats where it can be stored until eaten. Properties of the prey are also critical. If an organism can metabolize and eliminate a poison, biomagnification by a predator is likely to be minimal. Fat content in the body of a prey organism will also influence how much of the pollutant it can store. To say it is a complicated matrix would be a bit of an understatement!
Bioaccumulation. In practical terms, it can be difficult to distinguish dietary from non-dietary uptake. Consider, for example, the fish from the previous paragraph. Assuming they are swimming in contaminated water, the pollutant can enter their bodies through their skin (bioconcentration) and through the food they eat (biomagnification). The term bioaccumulation simplifies our lives as it refers to all pathways by which a pollutant ends up in an organism.
A word of clarification might be helpful at this point: the fates described here are not mutually exclusive. So, after a pollutant is released into soil, some of it can be degraded, some can move to the atmosphere, some to water, and some into organisms. Figure 15.8 presents a whimsical overview of the way a hypothetical soil pollutant is subject to many fates simultaneously.
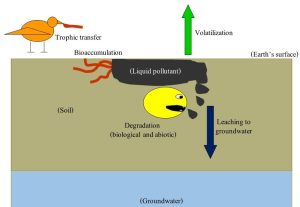
The relative importance of the possible fates, which can be represented in a four-compartment model, will depend on the chemistry of the compound as well as the properties of the environment into which it was dumped. Such a model visualizes each fate as a box, or compartment, in which some fraction of pollutant is contained. Note the distribution among the fates is likely to change with time as degradation and movement affect the amount and location of a pollutant in an area (Figure 15.9).
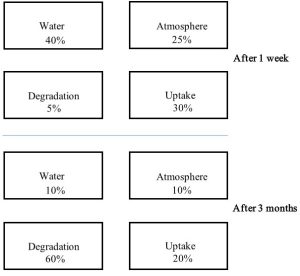
15.5.3. Effects of pollutants
Finally, we reach the portion of the environmental toxicology section in which we answer a crucial question: who cares? Environmental releases of poisons can lead to multiple adverse outcomes, only some of which we will address here.
Water pollution
Contamination of water, a problem we have encountered several times before (e.g., eutrophication), should stand out to you as a major threat to the health of humans and natural ecosystems alike.
Changes to individuals and populations
Uptake of pollutants by organisms (through diet or otherwise) can bring on many of the consequences we saw in Section 15.4 (harm to individuals due to cancer, nerve damage, and so on). Bioaccumulation (described just above near the end of 15.5.2) is especially worrisome because it can lead to very high levels of poisons in the tissues of organisms at the highest trophic levels (i.e., predators), even if the original level of contamination in water or soil was low. How can this be? Remember from Chapter 5 (especially Figure 5.18) that the amount of biomass in an ecosystem declines precipitously from the lowest to highest trophic levels. If the total amount of a pollutant within that system does not change (which occurs if the compound in question resists metabolism), the compound will be concentrated in less and less tissue (Figure 15.10). See Box 15.2 to read about a rather famous example of the effects of pollutant bioaccumulation.
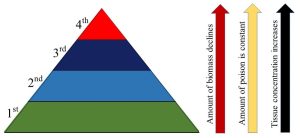
Box 15.2. The power of bioaccumulation
The phenomenon illustrated in Figure 15.10 was responsible for dramatic and well-known declines in the numbers of predatory birds such as the bald eagle in the United States during the 1960s and 1970s. As we understand now, widespread application of the pesticide DDT in and near waterways was the culprit. Very briefly, the target of DDT, malaria-spreading mosquitoes, died and ended up as food for small fish. Since DDT resists metabolism (it undergoes very slight alteration to something known as DDE and then persists for decades) and is readily stored in fat, it was a great candidate for bioaccumulation. Thus, the eagles and other raptors that ate the largest fish in the aquatic system received a very high dose of DDE, an endocrine disruptor that specifically affects eggshell formation. Eaglets (baby eagles) inside faulty eggs did not survive, and population sizes plummeted. As noted in Chapter 1, DDT was banned due to concerns raised by scientists like Rachel Carsen as well as its effect on bald eagle populations.
Two additional points that are screaming to be made will close out this, our last box together.
1. In addition to serving as a vivid example of the potential of bioaccumulation to harm organisms, the mosquito-fish-bird story brilliantly demonstrates the principle of selective toxicity. Note the different responses among the species: immediate death to mosquitoes (due to CNS disruptions), no apparent effect in fish, even at high doses, and reproductive failure in predatory birds.
2. Here is yet another great demonstration of one of our favorite and fundamental concepts, the principle of environmental unity. Who knew that attempts to prevent the spread of malaria would very nearly lead to the extinction of bald eagles?
Change in ecosystem structure and function
Structure. Organisms that are well adapted to their environments will survive, reproduce, and thrive in their ecosystems (review Chapter 1 and Chapter 6). If conditions change due to the presence of a pollutant, though, a heretofore successful population may lose its advantage and dwindle in importance. A poison that diminishes efficiency of reproduction, resistance to disease, coordination and strength, or other vital adaptations, is likely to lead to a change in the identity of dominant species in an ecosystem. For example, the release of endocrine disruptors into aquatic ecosystems can alter the male-to-female ratio of certain amphibians and reduce their ability to reproduce effectively. Not only is that particular species affected—worldwide populations of many amphibian species are declining[6]—but organisms at trophic levels above and below that of the amphibian can be put at risk. Certain fish species have similarly been affected by endocrine-disrupting chemicals[7].
Function. Important functions such as levels of primary productivity are often measured as endpoints (review Chapter 5 for more about ecosystem functions). If pollutants affect populations of plants or algae, rates of carbon fixation will decline, meaning a loss of nutrients for heterotrophs as well as, potentially, increases in atmospheric CO2 concentrations (see Chapter 14, the portions of section 14.2 about global climate change, for why such increases matter).
Development of an ecological gradient
A quirky outcome of environmental pollution can be a pattern of organisms around or near a source of contamination. Due to selective toxicity, organisms that are most resistant to the adverse effects of the poison will dominate the high-dose areas, whereas organisms that are more sensitive will only appear in relatively clean areas. In terrestrial systems, a distinct gradient can be observed, often featuring early stage successional species like lichen and grasses (see Chapter 5 to review ecological succession) growing in the harshest conditions and later stage species like shrubs, small trees, and then large trees, appearing in sequence as a function of horizontal distance from the pollution source (Figure 15.11).
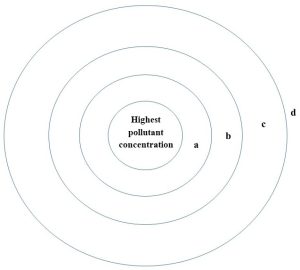
Disruption of human food production
The success of many crops depends on the actions of pollinating insects (e.g., honeybees). Thus, a chemical pesticide that damages an important pollinator has the potential to adversely affect food supply.
Human health effects due to consumption of contaminated food
Humans are not isolated from the effects of environmental contamination. In addition to the risk of polluted water noted earlier, we also can consume high-level predators and suffer the effects of bioaccumulation. In one high-profile case, the release of PCBs into the Hudson River in New York State (USA) in the 1970s led to bans and restrictions on consumption of fish caught in contaminated waters because of potential health risks associated with this group of persistent chemicals[8].
15.5.4. Management of pollutants
It should be obvious by now that humans have good reasons to minimize releases of pollutants into natural systems. Many laws are in place to encourage proper handling of toxic substances and limit environmental contamination (see Chapter 1, section 1.2 for some examples). However, poisons still end up in soil, air, and water. Some of the strategies used to clean up, or remediate, polluted environments are briefly described below.
Bioremediation
Here, the activities of organisms are harnessed to remove poisons from soil or water.
Soil. In some cases, we manipulate environmental conditions to stimulate indigenous microorganisms possessing the ability to degrade a pollutant. Sometimes, the necessary bacteria or fungi are not present and so are added to a contaminated site. This latter strategy also often requires manipulations, including the addition of limiting nutrients or oxygen, for it to succeed. Another common approach, phytoremediation, takes advantage of the ability some plants have to extract pollutants from soil.
Water. Very often, contaminated water is removed from its reservoir and cleaned elsewhere. Groundwater, for instance, may be pumped to the surface and put into contact with bacteria able to degrade the pollutant in question. See Figure 15.12 for a diagram of such a pump-and-treat system.
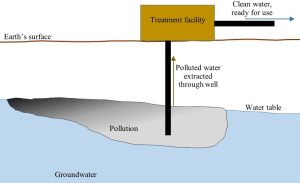
Chemical remediation
Instead of, or to supplement, the use of organisms, one of several non-biological strategies may be used to treat pollutants in soil. Among the chemical approaches are some that enhance the movement of contaminants out of soil and others that attempt to immobilize pollutants within the soil matrix so they do not contact organisms or move into water.
Excavation
When biological and chemical approaches fail, a far more expensive and disruptive solution is employed: all the contaminated soil in a field is dug out, loaded onto trucks, and hauled away. The many tons of soil can be incinerated at high temperature or stored in a landfill designed to contain hazardous waste. As you might imagine, the party responsible for funding such a project will try to avoid excavation by whatever means, including litigation.
Isolation
This final strategy does not actually remove the pollutant, rather, a fence and other steps are taken to restrict access to a contaminated site. Clearly, we would expect such a move only to serve as a short-term solution (review Chapter 1, section 1.2 and Figure 1.2 for an example), one intended to protect human and natural systems until a proper clean-up strategy is designed. But fences have been known to stay up for years or decades.
15.6. RISK ASSESSMENT
Risk assessors work to determine the potential adverse effects of any hazard on human health. So, why do we end the toxicology chapter on this note? Because the science of risk assessment often makes use of data obtained by toxicologists.
15.6.1 Terms and concepts
Like so many other fields, risk assessment uses its own language. Here we define those ideas that are essential to our brief look at the subject.
Risk
Risk is the probability that an adverse outcome will result from contact with a substance or device or as the result of participation in an activity.
Hazard
This term has two related meanings. First, it is an intrinsic property of a substance, device, or activity. In short, hazard is the potential something has to do harm. A chemical like hydrochloric acid (HCl) is a good example of a hazard because it can damage your skin. Importantly, the inherent danger posed by hazards does not change. Risk, on the other hand, depends on exposure and can vary a great deal. Let’s take another look at HCl to further distinguish between risk and hazard. If the acid is contained in a shatter-proof can that is stored behind a locked door that never gets opened, it poses no risk to you. Risk goes up only if your exposure to it goes up. Its hazard remains the same, though, whether or not you get near it as the acid always has the capacity to damage your skin. Second, the word “hazard” can refer to something that has an intrinsic, hazardous property and, thus, the potential to do you harm. This second meaning is extremely general, and includes everything from chemicals such as pesticides and drugs, to devices like artificial limbs and eye implants, to tools like step ladders and knives, to modes of transportation like cars and airplanes, to recreational activities like boating and amusement park rides, to athletic activities like skiing and baseball, to death-defying maneuvers like sky diving and bungie jumping. It is a very long list and quite a broad concept. Whatever the hazard, risk assessors calculate the probability of an adverse outcome as the result of exposure to it.
Safety
This is the inverse of risk, that is,
safety = 1 / risk.
If risk is the probability that you will be harmed from exposure to a hazard, safety is the probably that you will not be harmed by that same hazard. For instance, the risk of riding in a particular vehicle could be expressed as, say, 1 person out of every 20 ends up dead. Alternatively, that same ride could be said to be safe for 19 out of 20.
Adverse effect
Like response, an idea we encountered earlier in this chapter, adverse effect is defined as any undesirable / harmful outcome caused by exposure to a hazard. It is the endpoint measured by a risk assessor, the result of exposure to a hazard.
15.6.2. The process of risk assessment
Risk assessors seek answers to several questions as they analyze the likelihood that a hazard will cause harm.
Does the hazard cause the observed adverse effect?
Critically, we want to establish a mechanistic link between cause and effect. In the case of chemical compounds, data from humans exposed historically can provide some of the answer. Groups of people who worked with an industrial solvent for a decade or who smoked for 20 years, to provide just two of many possible examples, might be interviewed to connect observed health effects with past exposure. Often, tests using laboratory animals or individual cells also are used to help identify how a compound affects organisms.
What is the effect of dose on human response?
As above, human historical data as well as animal tests serve as good starting points here, but we will see in a moment that this question is not as easily—and reliably—answered as you might hope or expect.
What is the typical level of exposure to this hazard?
As we know, if the answer to this question is “none”, there will be no risk. Most of the time, though, we are worried about xenobiotics to which exposure is likely.
What is a safe level of exposure to this hazard?
Once we get answers to the three questions posed above, we get to the last step, the risk characterization. For a chemical compound like a food additive, we would see a simple-looking answer that describes a safe dose in terms of mg of the xenobiotic per kg of body weight. For example, the answer to the question posed here could be something like:
a safe daily human dose is expected to be 1.0 mg / kg ± 0.2 mg.
Very important is an expression of the level of uncertainty in our risk characterization. Here, it is included as the error term “± 0.2 mg”. Why is there so much uncertainty (20% in this case)? First, remember that all scientific measurements are expressed as a range due to random and systematic errors. But second, risk assessment adds its own sources of uncertainty that can produce rather large error terms. Critically, the principle of selective toxicity limits our ability to accurately predict individual human responses from animal dose-response data. To account for the differences in responses between and within species, risk characterizations tend to build in a lot of room for error, meaning that calculated safe human doses are commonly at least one hundred times lower than those seen in test organisms. The hypothetical recommendation of 1.0 mg / kg we saw above very likely would have been derived from an animal value of 100 mg / kg. The so-called safety factor, the amount by which the safe dose for animals is divided to establish a safe human dose (here it is 100), is used to better account for unavoidable uncertainty and reduce risk to humans.
The details of risk assessment of something other than a xenobiotic differ from the process shown here, but the guiding principles and goals are the same. For example, the likelihood of death due to riding in cars with and without seatbelts or the safety of a backyard trampoline could be calculated through analogous steps and analyses. We also can conduct ecological risk assessments to predict the potential adverse effects of a variety of human actions such as the construction of a new building, highway, or landfill on natural systems. Rather than endpoints related directly to human health, we would instead study loss of a keystone species, reduced primary production, or other ecological considerations described above.
15.6.3. Then what happens?
Results of studies conducted by risk assessors are provided to people who are at risk as well as those responsible for regulations on food, drugs, equipment, activities, and other hazards that could threaten human or environmental health. This latter group, known as risk managers, includes lawmakers who may accept the scientific data and craft legislation accordingly or ignore the recommendations due to multiple non-scientific factors including ideology, political expediency, and economic concerns.
THE CHAPTER ESSENCE IN BRIEF [9]
Myriad contemporary human activities produce potentially hazardous substances, although poisons have been a threat to organisms throughout history. Toxicology, the study of poisons and their possible effects, is founded on several important principles that influence the development, manufacturing, marketing, and use of drugs, pesticides, industrial chemicals, household cleaners and many other natural and synthetic materials.
Think about it some more…[10]
Is aspirin a poison or therapeutic drug? What about iron: harm or benefit? Ethanol? Cannabis?
How do antibiotics (Chapter 5) provide an example of selective toxicity?
Environmental scientists worry about the movement of poisons into the saturated zone. Briefly state WHY a compound that would otherwise break down in the unsaturated zone might persist in groundwater for an extended period nonetheless. As always, think like an environmental scientist as you ponder your answer (this sounds like another episode of connections between environments and organisms…).
Take another look at Figure 15.12. How do we know if the water exiting the treatment facility is clean enough? You might want to consider what we learned about water quality in Chapter 11 as you ponder your answer.
So, is everything toxic? Should you avoid contact with as much stuff as possible? What should you do with the information provided by toxicologists and risk assessors?
- For more information, see: U.S. Food and Drug Administration. 1999. Immunotoxicity testing guidance. www.fda.gov ↵
- U.S. Department of Health and Human Services, Agency for Toxic Substances Disease Registry. 2022. Per- and Polyfluoroalkyl Substances (PFAS) and Your Health. atsdr.cdc.gov ↵
- U.S. Department of Health and Human Services, Agency for Toxic Substances Disease Registry. 2011. Toxic Substances Portal. atsdr.cdc.gov ↵
- National Institute of Environmental Health Sciences. 2022. Endocrine disruptors. www.niehs.nih.gov/ ↵
- U.S. Department of Health and Human Services, Agency for Toxic Substances Disease Registry. 2015. Which Organ Systems are Affected by Toxic Exposures. atsdr.cdc.gov ↵
- Hayes TB, Case P, Chui S, Chung D, Haeffele C, Haston K, Lee M, Mai VP, Marjuoa Y, Parker J, Tsui M. 2006. Pesticide mixtures, endocrine disruption, and amphibian declines: are we underestimating the impact? Environ Health Perspect. Apr;114 Suppl 1(Suppl 1):40-50. doi: 10.1289/ehp.8051. PMID: 16818245; PMCID: PMC1874187 ↵
- U.S. Environmental Protection agency. 2022. Research on Detecting Endocrine Disrupting Chemicals from Animal Feeding Operations. epa.gov ↵
- U.S. Environmental Protection agency. 2022. Hudson River Cleanup. epa.gov ↵
- As you will find throughout this book, here is very succinct summary of the major themes and conclusions of Chapter 15 distilled down to a few sentences and fit to be printed on a t-shirt or posted to social media. ↵
- These questions should not be viewed as an exhaustive review of this chapter; rather, they are intended to provide a starting point for you to contemplate and synthesize some important ideas you have learned so far. ↵
An adjective that means of human origin. See Chapter 7 for details.
A general term indicating a measure taken to prevent something unwanted from happening. Note how prophylaxis is distinct from responding to something after it has occurred.
A process whereby a relatively clear aquatic system is transformed to first a murky then swampy then, potentially, a dry terrestrial system. It is caused by the accumulation of excess nutrients (nitrogen and/or phosphorus). See Chapters 4 and 11 for details.
A local air pollution problem generally seen in urban areas. Airborne products of fossil fuel combustion can get trapped near their sources and profoundly degrade air quality. See Chapter 14 for details.
A kind of energy that can travel at the speed of light from some source through air or even a vacuum. There are many different types of radiation. Some high energy radiation such as gamma rays can penetrate deep into objects (living and non living) and cause chemical changes. This kind of ionizing radiation can damage living cells. See Chapters 10 and 15 for more.
A general term that refers to clean up of water polluted with human excrement and other products flushed down toilets and other drains. Treatment involves a complex series of steps to minimize the presence of chemical, physical, and biological pollutants in water before it is released back to natural systems. See Chapter 11 for details.
A structure designed to contain waste. See chapter 13 for details.
A kind of radiation emitted by the sun. It is higher energy than visible light and can damage living cells (particularly cells of the skin). See Chapter 14 for more.
Refers to organisms that are too small to be seen without the aid of some kind of magnifying lens (e.g., a microscope). Includes bacteria, protozoa, certain forms of algae and fungi, and viruses. See Chapters 1 and 5 for more.
Refers to the many different kinds of microscopic organisms that are single celled and prokaryotic (i.e., do not possess distinct cellular organelles). Collectively, bacteria are extremely diverse, are the oldest organisms on Earth, and are critical to many environmental cycles and processes. See Chapter 3 for more.
A systematic strategy used by scientists to answer questions about the natural world. It is designed to facilitate objectivity and repeatability. The specific steps used by scientists different somewhat among disciplines, but they generally include some variation on: observation, hypothesis, experimentation, conclusion, repeat / refine hypothesis, share information with the scientific community. Chapter 2 contains a detailed description.
Refers to the smallest change an instrument can detect; put another way, the smallest possible measurement that can be made by an instrument. Contrast with accuracy and precision, and see more details in Chapter 2.
Refers to a group of organisms that are closely related enough that they can breed and produce fertile offspring. See Chapter 5 for more details.
A type of pesticide that specifically targets unwanted plants (i.e., weeds). See Chapters 9 and 15 for details.
The rigid layer found outside the cell membrane in the cells of plants and many microorganisms (but not animals). See Chapter 3 for more.
A biochemical molecule containing the code that makes every organism unique. See Chapter 6 for details.
Refers to a change from the normal or original genetic code. Mutations tend to damage or kill affected organisms but may impart new and helpful traits. See Chapter 6 for details.
The semi-permeable boundary that encloses all cells. Its chemical properties give it some control over what enters and exits a cell. See Chapter 3, especially Figure 3.9, for details.
In ecology, all of the living and non-living entities present in a defined space. See Chapter 5 for more.
Classed as eukaryotic microorganisms. Some are unicellular (e.g., yeast), many are multicellular (e.g., mushroom). They are are heterotrophic and tend to act as important decomposers in ecosystems. See Chapter 3 for more.
A pathway of the hydrologic cycle. Water flows across the surface of the Earth; it moves with gravity and follows the contours of the surface. See Chapter 4 for more.
A reservoir of the hydrologic cycle located below the Earth's surface. See Chapter 4 for details.
A pathway of the hydrologic cycle. Water flows vertically downward due to gravity. See Chapter 4 for more.
A process whereby a pollutant is carried vertically downward in moving water. It is of concern because it can lead to groundwater pollution. See Chapters 11 and 15 for details.
A general term that refers to the entry of a xenobiotic into an organism. See Chapter 15 for details.
In ecosystems, a group of organisms that all feed the same number of steps away from the original source of energy. For example, plants are all at the first trophic level because they get their energy directly from the sun, whereas deer and other herbivores are at the second trophic level because they get their energy from eating organisms at the first trophic level. Consult Chapter 5 for more details.
An interaction between organisms in which one is harmed and the other derives benefit. Specifically, a predator consumes a prey organism. See Chapter 5 for details.
A term referring to the total amount of living material in a place.
A potentially deadly disease caused by a kind of protozoan; that microorganism is itself spread by a certain type of mosquito.
A governing rule in environmental science that states, in short: all systems are connected to all other systems. The principle of environmental unity also implies that changes made to any system can bring about changes to other systems, even if those other systems are very distant from the initial change. Importantly, the resultant changes can be hard to predict and often are undesirable. See Chapter 1 for more.
In ecology, refers to the specific identity of organisms present in an ecosystem. This property varies widely among ecosystems. See Chapter 5 for details.
In ecology, a group of organisms that are all members of the same species. See Chapter 5 for details.
One of the processes carried out by organisms in an ecosystem. Important functions include C fixation, decomposition, and consumption. See Chapter 5 for details.
A type of organism that relies on preformed, organic carbon to meet its nutrient needs. Includes animals, fungi, and many bacteria. Heterotrophic an adjective. Compare to autotroph. See Chapter 5 for more.
A gas made up of one carbon atom and two oxygen atoms, it is one of the many forms carbon can take. This gas is the product of aerobic respiration as well as combustion of fossil fuels, wood, and other materials containing organic carbon. It is not directly usable as a carbon source but can be fixed into useable forms of carbon, for example glucose, by autotrophic organisms. See Chapters 4 and 14 for more.
In ecology, organisms that are most like pioneer species in their requirements. They tend to grow in relatively barren habitats. See Chapter 5 for details.
An example of a very close mutualistic relationship involving algae and fungi. This association is so evolved and so close that lichens take on a form that is distinct from that of each partner. See Chapter 5 for details,
An organism that is native to an area. An exotic organism would be the opposite, one that came into an area from an outside system. See Chapter 5 for more.
Refers to waste that poses more risk to human and environmental health than does domestic waste. Some waste from industry, hospitals, universities, and other facilities is classified as hazardous if it meets certain criteria. See Chapter 13 for details.
Cause measurements of a phenomena to be inaccurate in an inconsistent way (i.e., either above or below the actual value). For example, five different scientists might read a meter stick from five different angles and report five different answers; some of the answers will be too high and some will be too low. Contributes to scientific uncertainty. Compare to systematic errors. See Chapter 2 for details.
In science, cause all measurements of a particular phenomenon to be inaccurate in the same direction (i.e., either too high or too low). For example, if a balance is poorly calibrated, it could consistently read 0.2 g too high for all objects assessed. Contributes to scientific uncertainty. Compare to random errors. See Chapter 2 for details.
In ecology, an organism that plays a uniquely critical role in an ecosystem. If such an organism disappears, the affected system is likely to undergo dramatic adverse changes. See Chapter 6 for details.
Organisms that fix carbon for all members of an ecosystem; they are autotrophs. They also tend to convert non-biologically available energy into biologically available forms. For example, plants both fix carbon and convert the sun's energy to chemical bond energy. Consumers live off of the C and energy stored in primary producers. See Chapters 4 and 5 for more.