10 Energy Usage and Environmental Pollution
Jason Kelsey
So far, we have focused on the way energy enables processes fundamental to the maintenance of Earth’s natural systems, namely, the cycling of materials as well as the growth, survival, and reproduction of organisms. Now we consider how humans utilize energy in non-essential ways: to power the technology of modern societies and support the increasingly high standards of living described in Chapter 8. Given the large scope of this topic, Chapter 10 has been split into four sections. Part I is an overview and introduction, Parts II and III describe non-renewable and renewable sources of energy, respectively, and Part IV summarizes the present and possible future of energy.
Key concepts
After reading Chapter 10, you should understand the following:
- The three fundamental categories of human energy use
- That using energy is associated with unavoidable consequences
- How non-renewable and renewable energy sources are defined and distinguished from each other
- How different fossil fuels are formed by very slow, natural processes
- The environmental consequences of the widespread use of fossil fuels as energy sources
- How environmental scientists can predict the long-term supply of oil, natural gas, and coal
- The challenges, risks, and benefits of nuclear power
- The challenges and benefits of using renewable sources such as direct solar, wind, and moving water to generate power
- How biomass may be used as an energy source
- The distinction between primary and secondary energy sources
- How and why most of the world’s energy demands continue to be met by fossil fuels despite the availability of relatively clean renewable energy sources
- How energy supply and usage might look in the future
Chapter contents
PART I: OVERVIEW AND INTRODUCTION
10.1. Modern Societies Require Energy
10.2. Consequences of Energy Use
10.3. Renewability: an Introduction
PART II: NON-RENEWABLE ENERGY SOURCES
10.4. Non-renewables Dominate
PART III: RENEWABLE ENERGY SOURCES
10.5. Renewables Offer Alternatives
10.6. Primary and Secondary Energy Sources
PART IV: CLOSING WORDS AND FUTURE TRENDS
10.7. The Status Quo
10.8. An Alternative Future?
The Chapter Essence in Brief
Part I: OVERVIEW AND INTRODUCTION
10.1. MODERN SOCIETIES REQUIRE ENERGY
Energy conversions help us meet a variety of demands and perform many types of work (Chapter 4). Here we briefly introduce some important terms and describe different ways we use energy.
10.1.1. Three categories of energy usage
Electricity generation
Any device you plug into a wall outlet—your lamp, air conditioner, computer, mobile phone charger—is directly powered by this important form of energy. Although it occurs naturally (e.g., lightning), humans have devised ways to artificially generate and distribute usable quantities of electricity. At the most basic level, the process is straight forward: if magnets are rotated around a copper wire, electrons in the wire flow, that is, electricity is produced. The challenge, then, is to come up with a way to spin something so those magnets move as needed. As you might imagine, a wide array of possible solutions is available. You could employ your hamster’s wheel or your bicycle, for example, converting the energy in consumed food to electricity. Unfortunately, such small devices would yield limited amounts of usable power. Plus, of course, you and your pet rodent would tire after a while, halting the flow of electrons. These problems of scale and continuity of supply were overcome through the invention and development of the generator and steam turbine during the early 1800s and middle 1900s. Today, large power plants use the 19th-Century concepts of thermal generation to meet nearly all human demand for electricity. In simplified terms, these facilities convert energy stored in a fuel such as coal (there are others, as we will see) to heat, and that heat is used to boil very large quantities of water. Resulting high-pressure steam is directed at turbines and the magnets to which they are connected (Figures 10.1.a and 10.1.b).
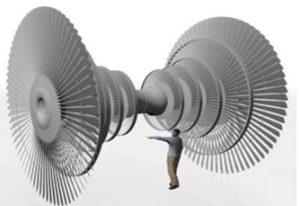
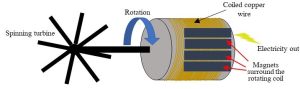
Steam is cooled, condensed, and then returned to the boiler to be turned into steam again. Given the large amounts of water used in the condensation process—it serves to lower the temperature of the steam—electricity generation plants are often built near natural reservoirs of the hydrological cycle (Figure 10.2).
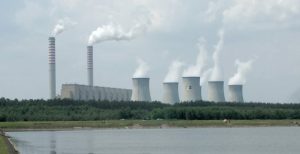
Electricity is then sent along wires into a larger network known as the power grid, or just “the grid” and distributed to homes, factories, and the like. The details of this last step are complex and not necessary for us to consider here; suffice it to say that electricity usage is far from uniform, changing with both location and time, and successfully meeting such heterogeneous demand requires extensive planning, monitoring, communication, and maintenance.
Note that the amount of power generated varies among facilities. Large coal-fired plants continuously meet the demands of hundreds of thousands of homes so long as the water is boiling and the turbines are spinning. Other types of plants can supply less or more power as a function of many variables we will explore later. Finally, recognize that the laws of thermodynamics (Chapter 4) govern and limit the efficiency of all energy conversions, including those that produce and distribute electricity. Among other important concerns, the amount of available electricity diminishes with distance from its point of generation—that is, more and more usable energy is lost as it moves along power lines to its point of use. So, electricity generated in, say, Arizona (USA), could not plausibly meet demands a few thousands kilometers away in the state of Vermont.
Temperature control
We generally strive to regulate the temperature of water and air in our homes, offices, and other interior spaces. When too warm, we turn to an electrical device such as an air conditioner or freezer to chill them. If cooler than we would like, though, we use hot water heaters or furnaces to convert chemical energy to heat and raise their temperatures. The process resembles that of electricity generation in that fuel is burned, often natural gas or oil, but here we do not go to the next step of spinning a turbine. Instead, hot water and / or air is distributed to a building’s climate control and plumbing systems, often with the aid of an electricity-powered blower or analogous machine, as desired by human users.
Transportation
The ability to reliably and regularly move people, animals, food, and other objects both short and long distances has become central to the functioning of modern societies. As is well known, coal and petroleum products have served as portable fuels to power nearly all this work—transportation—since the beginning of the Industrial Revolution and appear poised to continue in that role for some time (much more about these energy sources is described in Part II, below). For example, when gasoline is burned in an internal combustion engine, chemical energy is released and converted into kinetic energy: pistons move up and down, wheels spin, and a motor vehicle is propelled along the street.
10.1.2. Total energy vs. electricity
A word of caution is in order at this point. You should note the potentially confusing distinction between total energy, which includes electricity, heating, and transportation, and electricity alone. Be on the lookout for the two terms as we proceed, and remember that they do not refer to the same thing.
10.2. CONSEQUENCES OF ENERGY USAGE
Energy conversions to meet demands for electricity, temperature control, or transportation initiate processes that lead to two consequences, one of which is highly likely, and the other of which is unavoidable.
10.2.1. Environmental degradation
Although the nature and severity of it varies, this consequence is virtually certain. For example, in addition to the large amounts of useable energy they release, both coal combustion and nuclear fission produce harmful products. Even the use of energy sources generally regarded as clean, such as solar and wind, has some environmental costs. Details about these and other adverse consequences linked to power generation will be presented in Parts II and III of this chapter.
10.2.2. Loss of usable energy
Substantial amounts of energy must be invested—never to be used directly by us—in the interest of power generation. Two phenomena are responsible for this second, inevitable, consequence.
Technology used to obtain energy needs power to operate
Speaking rather informally, it takes energy to get energy. Keep in mind that the machinery necessary to acquire, process, and transport our various energy sources must be powered somehow. For example, drills, pumps, and marine tankers, just some of the technology used in the exploitation of fossil fuels, generally rely on the combustion of fossil fuels themselves. Although our goal is to capture as much net useable energy as possible, losses are unavoidable. Importantly, the worth of a particular resource is often judged by the relationship of the amount of energy needed to obtain it to that provided by it. No fuel will produce more energy than is invested in it, but efficiencies do vary substantially.
Energy conversions are inefficient
Recall from our consideration of the second law of thermodynamics in Chapter 4 that every energy transformation leads to a reduction in the amount of usable energy in the universe. So, when the potential energy stored in a fuel such as gasoline is converted to the kinetic energy of a moving car, some amount of low grade heat is always released (review Box 4.6). Powering technology, therefore, will necessarily reduce the amount of high-grade energy available in the future. The practical relevance of this reduction varies considerably among energy sources as some are so abundant that usage has a trivial and irrelevant effect on their availability whereas others are appreciably diminished when they are used to do work. We will hear more about the importance of this distinction below.
10.3. Renewability: an Introduction
We group energy sources according to the effect current usage has on future supply (as noted just above), or on what is commonly known as their renewability.
Non-renewable sources. The supply of these sources is substantially reduced when they are used to do work. Once again, systems analysis is useful here: since the rate of usage, or output, far exceeds that of input, or formation, the quantity of non-renewables in Earth’s systems decreases (Figure 10.3).
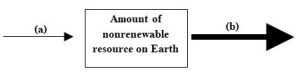
Importantly, although it is true that materials such as coal and petroleum are replenished by natural processes, their rate of replacement is far too slow to keep up with demand. We will focus a great deal of attention on these and other non-renewable energy sources in Part II of this chapter.
Renewable sources. A commonly used way to assess renewability is to determine the length of time required to replenish a resource after the high-grade energy in it has been degraded. If it can be replaced in a period that is short enough to be useful and relevant for humans, then it is counted in this group. Note that renewability does not imply the energy is somehow recycled or reused (in what would be a violation of the laws of thermodynamics), rather, high-grade energy degraded to low-grade energy is replaced by an additional quantity of high-grade energy. It is also appropriate to classify a source as renewable if its supply is sufficiently large that humans could not plausibly deplete it. Consider the sun, which easily meets this second standard of supply vs. usage. It will continue to release energy so far into the future, for several billion more years, that it might as well be infinite and inexhaustible as far as we are concerned. Solar, wind, and hydrothermal energy are among the renewables we will study in some detail in Part III.
Part II: NON-RENEWABLE ENERGY SOURCES
10.4. NON-RENEWABLES DOMINATE
These have provided the bulk of our energy since the beginning of the Industrial Revolution of the 1800s. In this section we will explore advantages and disadvantages of each, paying close attention to three considerations: how it is formed and / or used to provide power, the environmental costs associated with it, and how much demand it can meet.
10.4.1. Fossil fuels
These have well-known economic and cultural importance. They are energy rich, portable, and versatile enough to provide power in different ways. Note they are naturally occurring, having been derived from organisms that died millions of years ago (Chapter 3). You should also realize that their ultimate source of energy was the sun because the relevant organisms lived in ecosystems powered by photoautotrophs (Chapter 5). The fossil fuel group includes crude oil, natural gas, and coal.
Crude oil and natural gas
These first two are fluids with similar chemistry but different physical properties. Each is composed primarily of carbon and hydrogen atoms (i.e., they are called hydrocarbons—see Figure 10.4), however, crude oil is a dense, thick, tarry liquid, whereas natural gas is a light, gaseous substance.
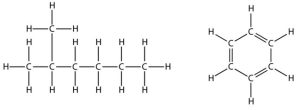
The term petroleum is often used as a synonym for oil, although some classify natural gas as a petroleum product as well. They are paired in our discussion here, with “petroleum” used to refer to both, because they generally form under the same extremely specialized and unusual conditions.
Formation. The story of oil and gas begins with tiny plankton—primitive plant- and animal-like organisms—living in surface waters of ancient oceans. After they died, decomposition converted all but a small fraction of their remains to inorganic products like carbon dioxide. The organic molecules that persisted sank and mixed with inorganic materials (e.g., weathering products like sediments) in low-oxygen environments on the seafloor. Note that this lack of O2 gas limited aerobic organisms that would otherwise quickly break down the accumulating cells. Burial under additional sediments pushed the materials deeper into the lithosphere, subjecting them to elevated temperatures (as they moved toward Earth’s hot interior) and pressures (squeezed together by overlying layers of sediments). Metabolism by anaerobic bacteria (Chapter 1) likely acted upon them as well. At a few hundred meters depth, the biological materials became a waxy intermediate product called kerogen. Beginning around 2 km, the kerogen underwent conversions to crude oil. Increasing temperatures and pressures with continued burial yielded natural gas. Realize that at this stage the materials were not concentrated in some sort of underground pools just waiting for humans to find them, instead, they were dispersed in the pores of sedimentary rocks (Chapter 3) located several kilometers below the surface. It took millions of additional years for the fuels to move upward from what are known as their source rocks to shallower locations where they collected in reservoir rocks. Why did they move against gravity? Most petroleum is less dense than solid rocks and will rise through cracks and pores, assuming such openings are present, toward Earth’s surface. In fact, oil and gas continue to move up until and unless they encounter a barrier, or oil trap, an impermeable geologic structure through which fluids cannot readily pass. For example, a fault (Chapter 7) can trap large amounts of petroleum and lead to the formation of an economically important oil field. When all was said in done, on the order of 200 million years elapsed between the death of those ancient plankton and the appearance of useable quantities of petroleum. Figure 10.5 presents an overview of the formation of oil and natural gas reservoirs, including an example trap, and Box 10.1. provides a bit more information about the biological origins of oil.
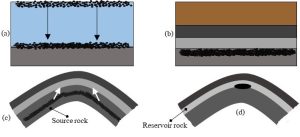
Box 10.1. Not dinosaur fossils!
Oil is often mistakenly thought to be the remains of dinosaurs, but alas, the real story of its origins is quite different. Most of the oil we see these days got its start hundreds of millions of years ago, before those famous extinct animals even roamed the Earth. Chemical analysis of petroleum in the lithosphere confirms that it is made up of the remains of tiny plankton that once lived in ancient oceans. Perhaps this is a less glamorous story than we would like, but it is one supported by data.
Note that in some cases oil does not encounter an effective trap during its upward journey but slowly bubbles out, or seeps, into ocean waters from the seafloor. During millions of years, certain marine bacteria have adapted to eat this oil that enters their environments! Scientists take advantage of these microorganisms to clean up spills, as described in more detail in Box 10.2 (upcoming).
Environmental costs. Each of the many steps required to bring oil and gas from their natural reservoirs to the places they are used by humans causes degradation of natural environments.
Exploration
Oil and gas are found in places subjected to the conditions that led to the formation and accumulation of these fuels hundreds of millions of years ago. The challenge, then, is to find those reservoirs from which useable quantities can be extracted (next step, below). Several tools are used to explore. First, a study of the geology of an area is essential. Since we broadly understand how oil is produced, we can examine data to narrow down locations that are likely candidates. For example, places such as present-day Saudi Arabia and Texas were once environments conducive to petroleum formation. Second, satellite images and maps can be used to search for surface features associated with reservoirs. Finally, specialized instruments allow a direct study of rock layers below Earth’s surface in areas thought to harbor oil: the behavior of artificially generated vibrations sent underground can indicate whether the drilling of exploratory wells is warranted. If oil is discovered, an above-ground ecosystem can be profoundly altered as land is cleared and roads, buildings, and more wells are constructed. When reservoirs are beneath the ocean, analogous changes will occur as oil platforms are built.
Extraction
Removal of petroleum from reservoirs—known as petroleum recovery or extraction—is accomplished through several different methods, and each can cause appreciable environmental degradation.
Conventional oil drilling. This is currently the most widely used technique. Here, large metal drills are driven into the Earth, producing a vertical hole that leads to underground reservoirs. The opening is then subsequently lined with pipe and other materials. Such wells can be built in marine or terrestrial systems, using oil rigs or oil platforms, respectively (Figures 10.6.a and b).
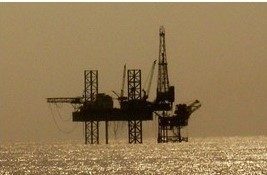
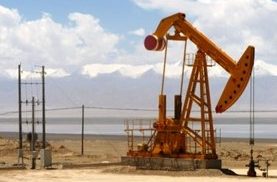
The process is complicated for many reasons, including the fact that the methods used to obtain oil from any given well generally must change with time. When a drill first penetrates a new reservoir, a tremendous amount of pressure can be released, sending oil gushing up the pipe toward the surface. During this early stage, oil is obtained relatively easily because it is not tightly bound in reservoir rocks. However, only about 10% of the fluid in a typical well can be recovered through simple pumping. Large amounts of energy (and money) are required to remove oil that is locked up in pores and other places. In the end, it is not unusual for over half of the oil in a reservoir to be left behind because it is too costly to obtain it.
Extraction from either land- or sea-based wells is linked to several adverse environmental consequences. First, oil can spill into natural systems due to damaged equipment or errors. These events can be particularly problematic when pipes or pumps lie deep below the surface of the ocean. The case of the Deepwater Horizon accident of 2010, which occurred under about 1500 meters (~1 mile) of water in the Gulf of Mexico, provides an extreme example of the potential dangers of oil drilling. An explosion killed eleven people, sank the platform, and ultimately generated a large leak on the seafloor—some 15 million liters (~4 million gallons) of oil escaped into the water during the nearly three months that passed before the gushing well was capped[1]. Spills of this kind can cause substantial amounts of damage to environmental and human systems (see Box 10.2).
Box 10.2. Marine oil spills: consequences and clean up
1. Adverse consequences.
(a) Petroleum can kill fish, mammals, seabirds, and other organisms living in and near the water. It is poisonous and can also reduce the insulating capacity of feathers, causing coated waterfowl to freeze to death (Figure 10.7, left)
(b) Spills can create public-relations nightmares for responsible parties, as when they leave beaches coated with oil (Figure 10.7, right).
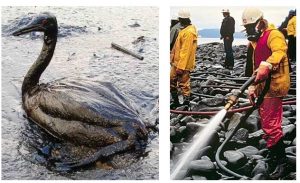
(c) Oil spills cause human suffering. Substantial economic losses can affect individuals and communities that rely on ocean resources for their livelihoods. The spill associated with the Deepwater Horizon explosion (described in the main text), damaged fisheries, including those that harvest oysters.
2. Can they be cleaned up? As noted in the main text, petroleum hydrocarbons can be decomposed by marine microorganisms adapted to use them as nutrient sources. Some fraction of spilled oil is, therefore, broken down by natural processes. However, since the amount of oil released at one time by a large accident can overwhelm ocean systems, human intervention is generally needed to reduce adverse consequences. Time is often of the essence, because we want to keep spilled oil from spreading over the water’s surface and moving onto beaches where its removal is even more difficult. Several remediation (i.e., clean-up) strategies may be used.
(a) Containment. As soon as is possible, efforts are made to slow the spread of oil from its source (e.g., a damaged well or tanker). Floating barriers known as booms (Figure 10.8) are often set up at the periphery of a spill to reduce damage to both water and beach ecosystems.
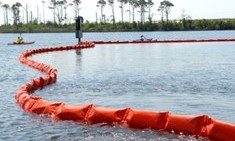
Although containment is clearly just a short-term solution, it can buy clean-up crews some time to deploy other strategies to eliminate oil.
(b) Physical removal. In effect, floating oil is scooped off the surface of polluted water using devices called skimmers. Skimming is a slow process but can be effective in reducing the amount of oil that ultimately moves onto beaches. High-pressure washers have been used to blast oil from rocks and sand, but their hot water tends to kill many of the bacteria that would eventually be able to remove much of the oil if allowed time to do so.
(c) Breakdown. Naturally occurring microorganisms with the appropriate adaptations can be harnessed to clean up oil. The extent to which this strategy is effective depends on many difficult-to-control variables. If the ocean is very cold (as in the arctic), nutrient poor, or otherwise hostile, bacteria able to break down oil in idealized laboratory experiments might not do so in a real-world situation. Scientists continue to study ways to enhance the survival and activity of microorganisms to help remediate ocean oil spills. We will see more about the use of microorganisms to breakdown environmental pollutants in Chapter 15.
Second, the drills, pumps, and other machinery required to recover oil release air pollutants because they are powered by fossil fuel combustion. Third, existing ecosystems and landforms are disrupted as space is made for oil rigs, roads, pipelines, and the rest of the infrastructure associated with oil drilling. Land subsidence can even occur in areas that lie above wells, because, in some cases, critical support for Earth’s surface is lost when large amounts of oil are extracted (Figure 10.9). Buildings can be damaged by shifting ground, and inundation by water can cause widespread flooding.
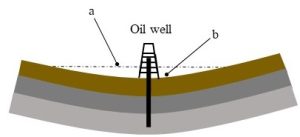
Conventional natural gas drilling. Oil and natural gas are often found near each other and are recovered from porous rocks through similar methods. They are also associated with many of the same environmental concerns: air pollution, ecosystem disruption, and changes to landscapes. Since it is gaseous, though, accidental releases of natural gas cause air pollution to a far greater extent than do oil spills. As we will see in detail in Chapter 14, methane (an important component of natural gas) plays an important role in raising Earth’s average temperature and changing its climate.
Unconventional methods. For various reasons, much of Earth’s petroleum does not lend itself to recovery through conventional wells. In such cases, one of several alternative extraction techniques can be employed. Although these methods have the potential to greatly increase the amount of oil available to us, they generally are more expensive than conventional ones and present their own environmental challenges. Commonly used unconventional methods include mining from oil sands or oil shales and hydraulic fracturing. We will look only at the last technique on this list.
Hydraulic fracturing, commonly referred to as fracking, is used to free up so-called tight petroleum that cannot be extracted by conventional drilling methods. In these deposits, oil and gas—in some cases, enormous quantities of them—are trapped in individual, isolated pores within shale (a type of sedimentary rock). Importantly, these openings are not connected to each other, meaning that fluids do not flow through the rocks efficiently enough to justify the expense associated with conventional pumping (Figure 10.10 distinguishes porosity from permeability, two properties that influence storage and extraction of oil and gas within rocks).
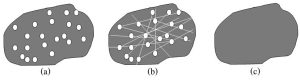
Fracking was developed to increase permeability in such deposits. In short, fluids consisting of water, chemical compounds, and sand-like materials are pumped into reservoir rocks at such high pressure that new cracks and channels form. After the rocks have been fractured, the contents of those many pores flow toward each other and become concentrated in relatively small areas; rigs can then pump the petroleum to the surface. In recent years, extraction efficiency has been further improved through horizontal drilling. Here, a vertical well pipe is curved so it runs in a direction roughly parallel to the Earth’s surface through reservoir rocks (Figure 10.11).
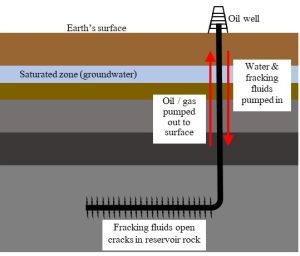
Several environmental concerns unique to fracking have made it a controversial technique. First, large volumes of water are used to break up rocks and increase permeability. In places like Pennsylvania, where water is relatively abundant, high usage is generally not viewed as limiting or even much of a concern. However, fracking in Texas and similarly dry areas could further strain already limited water supplies. Second, the fluids injected into rocks to increase permeability could spill onto the surface or leak from broken pipes and move into groundwater (Chapter 4 and Chapter 11), substantially degrading an important source of drinking water for many people. Finally, there have been suggestions that methane gas released by fracking can make its way into the water supplies of nearby houses. A quick internet search can even reveal sensationalized video images of homeowners igniting their sink faucets! Many questions, both scientific and legal, have arisen about the possible link between fracking and what appears to be combustible water, but definitive answers have yet to be found.
Transport
Transport is needed at two different stages of the petroleum cycle: to move materials pumped from wells to refineries (below) for processing and conversion into various fuels, and to move the products of refining to the places at which they will be used. It is important to recognize that, when initially extracted from the Earth, petroleum is generally not in a form that is directly useable by humans. For example, crude oil is the material obtained through conventional oil rigs. This viscous, tarry substance can rarely function as an energy source for electricity generation, transportation, or heating. Crude must first be transported from wells to facilities at which it is converted into separate fuels (more on these conversions is presented below). For both crude oil and refined fuels, one of four transport methods are common.
Pipelines. Essentially large metal tubes that can extend for thousands of kilometers across and under Earth’s surface, pipelines are commonly used to transport petroleum (Figure 10.12).
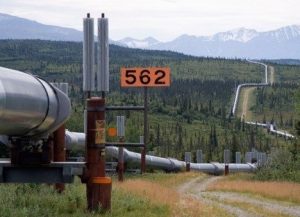
Two important environmental concerns arise from pipelines. First, land is cleared and ecosystems disrupted when they are constructed. Among other problems, movement of migratory land-based organisms can be interrupted by the presence of these large barriers (recall habitat fragmentation in Chapter 6). Second, ruptures in oil pipelines can cause the same kinds of consequences seen above in the discussion of oil extraction, namely pollution of water and soil.
Pipelines are the dominant means by which natural gas is transported from wells to processing plants and users (as we will see, other methods also play important roles in the transport of oil). Large-diameter tubes move it long distances, and then smaller ones carry it into homes and businesses. The need for pipelines has placed limitations on how much gas can be moved among continents (i.e., they are not conducive to transporting material across oceans), meaning that it cannot be as easily distributed from producers and consumers as can oil. However, when it is cooled to very low temperatures, it becomes a liquid that can be shipped via vehicles (like trucks, trains, and marine tankers). Increasing interest in this liquefied natural gas (LPG) could change global markets, a point we will briefly revisit again later.
Rail cars and on-road trucks. These means of transport carry less oil than do pipelines and have considerably less capacity than do marine tankers (next), but they still are used in many cases. Trucks are valued for their flexibility—they obviously can deliver directly to many more places than can pipelines. The risks they pose are like those we have seen already: air pollution from fossil fuel combustion and human and environmental damage due to accidental releases.
Marine oil tankers. When over-land methods are impractical, large ships are used to move oil across oceans (Figure 10.13).
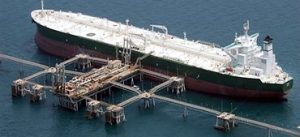
Just one of these tankers can hold up to 2 million barrels[2] of liquid (note that one barrel contains about 169 liters, 42 gallons, of petroleum), so, if all goes as planned, this means of transport is relatively cost efficient. Unfortunately, though, tankers have been known to crack and release their cargo into seawater. Most of the time, such accidents involve small quantities of petroleum, but since 1969 there have been 44 events releasing 10,000 or more barrels each (about 1.6 million liters) worldwide[3]. The consequences of spills depend on the location in which the oil is released, but damage to both natural coastal ecosystems and human welfare is common (review Box 10.2 for more).
Refining
Crude oil extracted from the lithosphere is taken to large facilities known as refineries where it is treated in a multi-step process to produce different fuels. Refining starts when crude is heated to yield several separate fractions (i.e., individual fuels). Relatively light gasoline, kerosene, and some diesel fuels are among the outputs collected after this stage. Next, the heavier fractions left from step one are subjected to high pressure, further heating, and various other processing to generate additional gasoline and other valued commodities. Finally, treatment to remove impurities and otherwise prepare the refined products for sale and use is carried out as needed.
In the end, for every barrel of crude that enters an oil refinery, about 20, 12, and 4 gallons of gasoline, diesel fuels, and jet fuel, respectively, come out (the remainder becomes asphalt and other petroleum products)[4]. Figure 10.14 summarizes refining and its products.
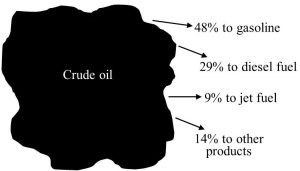
Refining causes some important adverse environmental effects. Many air pollutants are routinely emitted, including heavy metals, dust particles, carbon dioxide, sulfur dioxide, nitrogen oxides, and others (we will see more about sources and effects of air pollution in Chapter 14). Hazardous waste regularly enters waterways adjacent to these facilities as well. Moreover, refining is very energy intensive, and a lot of fossil fuels are burned to carry out the various stages of it. Accidental releases from refineries can also damage natural systems, and air and water pollution are ongoing concerns (Figure 10.15). Issues related to transport are applicable here as well because the finished products must be moved to their points of sale and use.
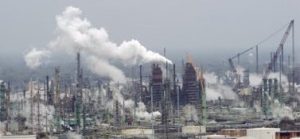
You should be aware that natural gas extracted from wells does not undergo the same kind of refining as crude, although it still must be processed to some extent before it is usable. Various hydrocarbons, water, and other impurities are removed at both the site of a well and remote treatment facilities to yield a product that is nearly 100% methane.
The outputs from refineries are transported to places like gasoline stations and power plants. A great deal of energy is released when fossil fuels are then burned and converted to products—after all, that is why these materials are so prized—but, their use generates several air pollutants as well.
Carbon dioxide. Upon combustion, nearly all the organic carbon in petroleum ends up bonded to oxygen atoms to form carbon dioxide gas. On the order of 8,900 grams, or 19 pounds, of CO2 is released for each 3.8 liters (1 gallon) of gasoline burned (and about 511 liters—135 billion gallons—were burned in the United States during 2020)[5]. Note that, when compared on the basis of equivalent amounts of energy yielded when combusted, diesel fuel releases about 5% more and natural gas releases about 30% less CO2 than does typical gasoline[6]. This gaseous product presents environmental concerns not because it is directly toxic to humans (although in very high concentrations it can push oxygen away and cause asphyxiation), but because it is one of several important greenhouse gases that contribute to global warming and climate change (Chapter 14).
Other carbon products. The remaining C atoms get incorporated into carbon monoxide molecules, methane, and various hydrocarbons. The first on that list of three, CO, is poisonous to humans and other organisms and can undergo chemical transformation to CO2. Methane is of concern because, like CO2, it plays an important role in the process of global warming that we will explore in Chapter 14. Recall that “hydrocarbon” is a general chemical term that refers to any compound made up of C and H atoms. Small amounts of many different hydrocarbons are produced when fossil fuels are combusted, and they contribute to a phenomenon known as smog (also coming up in Chapter 14).
Nitrogen and sulfur gases. Nitrogen oxides, often represented by “NOx” to suggest that the number of nitrogen and oxygen atoms can differ among compounds, and sulfur dioxide, SO2, also can exit the tailpipes of motor vehicles (combustion of diesel fuel is a particularly important source of sulfur gases). Many of these gases may be transformed into acid precipitation and are important components of smog (again, more about both consequences will be presented in Chapter 14).
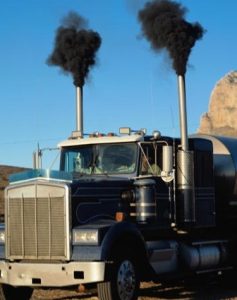
Particulates. These dust-like physical products are often visible as dark-colored clouds in the exhaust of diesel vehicles (Figure 10.16). Concerns about particulate matter include respiratory and other health effects among exposed humans, reduced visibility in affected air, and pollution of water and soils into which the solids ultimately settle.
Mercury. Since petroleum often contains mercury, combustion releases this toxic metal into the atmosphere. Through various mechanisms it is eventually deposited into soil and water where it can damage individuals and ecosystems. In a related note, lead was once added to gasoline and emitted like mercury when fossil fuels were burned, but, starting in the 1970s, it was phased out of fuels used by passenger vehicles in the United States.
Meeting present and future demand. It would not be an exaggeration to say the industrialized world is dependent on petroleum. In 2021, oil and natural gas met approximately 36% and 32%, respectively, of the total demand for energy in the United States (worldwide, the numbers are similar)[7]. Since humans consume them faster than they can be naturally replaced, these levels of use are not sustainable, and, if nothing changes, the planet will be effectively emptied of oil and natural gas at some point in the foreseeable future. A universally agreed upon answer to the question “just when will we run out of petroleum?” has proven to be elusive, however, with estimates ranging from as low as a few decades to as high as a few centuries. These disparities arise from several sources, including differences in assumptions about the size of conventional and unconventional reservoirs, future demand for fuels, and the future costs and availability of alternative energy sources (more about these later, in Part III). Here, we briefly explore some important facts about production and use of petroleum and then return to the question of how long our supply might last.
Calculating supply: reserves vs. resources
Not all petroleum on Earth is equally accessible. A portion of it can be readily extracted using conventional methods, but much of it is not so easily obtained. In the latter case, one of the unconventional methods noted above may be tried, however, some material is so tightly held within the lithosphere that it cannot be removed with currently available technology. Furthermore, most scientists assume some modest quantity of petroleum is yet to be discovered, and the search for new oil fields continues. Since exploration is generally informed by knowledge of geology and predictions about probable locations of untapped reservoirs, estimates about how much more we can expect to find are often made. Combining considerations about relative accessibility with informed guesses about future discoveries, we have established different ways to quantify and report supply. Quantities of petroleum (and for that matter, any natural material of interest) that have been located and can be extracted in an economically viable way are classified as proven reserves. On the other hand, the term resources refers to known reserves plus material that is more difficult to recover for one or more reasons. For example, oil that has been discovered but is stored such that it is either currently impossible (due to technological limitations) or too expensive (i.e., it costs more money to produce than it will return when sold) to extract would be counted here. Oil that is thought to be present in an area without confirmation that it really exists is also included with resources. The word “resources” clearly represents a far more optimistic appraisal of supply than does “reserves”, so we should interpret and use the two terms with great care.
Petroleum distribution is heterogeneous
Oil. Due to past differences in environmental conditions around Earth, the current availability of oil and natural gas is unequal. It would be fair to say, in fact, that petroleum is concentrated in a relatively small number of nations. An estimated 80% of conventional oil reservoirs (i.e., proven reserves), about 1.2 trillion barrels, are controlled by the Organization of the Petroleum Exporting Countries (OPEC)[8]. This group is made up of about fourteen nations (the number can change from time to time) from the Persian Gulf region, northern Africa, Latin America, and Asia. Saudi Arabia and Venezuela, two members, together hold over half of OPEC’s oil. On the other hand, most of the world’s unconventional supply is in the United States, Canada, Venezuela, and Russia. Estimates vary, but another 2 trillion barrels (possibly much more, according to some rather sanguine calculations) could be held in these difficult-to-exploit reservoirs. Recent studies even indicate that, when all resources are included, more oil is present in the United States than in any other country[9].
Conventional reservoirs dominated world supply for most of the past century, and combustion of them has eliminated nearly 1 trillion barrels of Earth’s supply (you should know there is some debate about the size of that figure). However, with the development of new technology, oil from unconventional reservoirs, even some that has historically been inaccessible, is now extractable and relevant. In the U.S. alone, the percentage of crude oil production from hydraulic fracturing and related methods rose from essentially 0 in the year 2005 to 48 in 2017, and the amount of oil coming from those unconventional sources is predicted to increase by a factor of two or three in the next thirty years[10].
Natural gas. As with oil, reservoirs of this important energy source are unequally distributed: about two thirds of the 200 trillion cubic meters (tcm) of proven natural gas reserves are controlled by just five countries: Russia, Iran, Qatar, the United States, and Saudi Arabia[11]. Relatively new technology allowing extraction of tight gas has greatly increased the estimated size of Earth’s natural gas resources by about three fold (i.e., including all recoverable reserves and resources). The continued high cost of exploiting these unconventional reservoirs, though, is an important factor limiting long-term supply.
Demand
Oil. Demand for oil has risen during the past several decades and is likely to continue that trajectory for the next several. Humans use around 100 million barrels per day (37 billion per year) and that number is projected to increase in coming years, especially as usage in China, India, and other nations in the developing world goes up[12].
Natural gas. Several factors, among them worries about crude oil supply, reduced air pollution when it is burned instead of oil, and the emergence of new approaches to transport that allow intercontinental distribution of natural gas to regions that lack significant local suppliers (using LPG), have stimulated a doubling in global consumption since 1980[13] (current demand is about 4 tcm and, despite current short-term declines in demand, likely to rise in coming years[14]). Recent discoveries of vast reservoirs of tight gas, including those in the Marcellus Shale deposits of New York, Pennsylvania, West Virginia and a few other eastern U.S. states, along with the Barnett in Texas and elsewhere, have also stoked usage. This fuel has become increasingly important in electricity generation, heating, and transportation, and that trend is likely to continue. In just one example, power plants that rely on coal as an energy source are steadily being replaced by those that use natural gas because the former emit more air pollution than the latter (will we see more about coal below).
How long can demand be met?
Oil. We now attempt to address the seemingly straight-forward, yet difficult-to-answer, question we posed at the beginning of this section. How much oil remains on Earth is hotly contested and depends on both who you ask and how they measure. The most conservative, if widely accepted, assessments count only the so-called proven reserves of oil, or about 1.6 trillion barrels[15]. Now, if we divide the amount of oil left by current demand (1.6 trillion or 1,600 billion barrels / 37 billion barrels per year) we can conclude it will run out in 43 years. There are two caveats to keep in mind here: 37 billion barrels a year surely underestimates future demand, and total supply is likely higher than 1.6 trillion barrels (remember, some additional untapped reserves probably will be discovered and we can count unconventional reservoirs). Thus, this number may represent the low end of future availability. Less conservative estimates add oil believed to be tied up in difficult-to-access reservoirs, as well as undiscovered crude, that is, include all oil classified as proved, probable, and possible (three categories ranging from most certain and accessible to least). Taking those less-reliable pools into account, the total amount of oil left could range from 2 – 4 trillion barrels, or last up to about 130 years. Finally, some extreme estimates envision 10 trillion additional barrels of unconventional oil (yielding a theoretical sum of 11.6 trillion), meaning that present-day demand could be met for well over 300 more years. Consult Box 10.3 for more about predicting the future of oil and the concept known as peak oil.
Box 10.3. Peak oil?
In 1956, an American geologist named M. King Hubbert made an evidence-based prediction about future supply and demand for oil. He observed that oil will progress through stages of increasing discovery, production, and use that inevitably leads to depletion and ever-declining availability. His model, represented in what is now known as a Hubbert Curve (Figure 10.17), is based on several assumptions. First, in the early years of the industry, discoveries of petroleum reservoirs outpace the use of oil. Then, demand increases as supply increases until production reaches it maximum, or peak level. After the peak, supply cannot keep up with demand and prices increase irrevocably. Eventually, the amount of oil on Earth diminishes to levels so low as to be irrelevant.
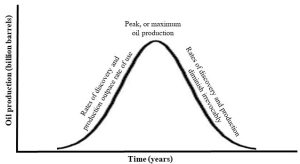
Hubbert was partially correct when he predicted that petroleum would peak in the 1970s in that conventional oil production in the 48 contiguous U.S. states did reach its maximum then. But he did not account for oil from places such as Alaska, OPEC, and Russia, nor did he anticipate unconventional oil. Many have discounted his model completely because it failed to predict the correct year of peak oil. Others take it more seriously, noting how it reasonably describes the fate of oil and any finite resource. In other words, although the absolute timing of his prediction was clearly off, the overall trend of Hubbert’s curve fits our basic understanding of what will happen if we continue to consume oil far faster than it is replaced by natural processes.
The story gets quite a bit more complicated when we consider how price affects supply. For instance, increases in the cost of petroleum—vividly manifested by soaring prices at gasoline stations—during the 1970s led to economic turmoil for nations that imported much of their oil. By and large, short-term demand during these periods of rapid change remained constant; people continued to buy more expensive petroleum products because they simply needed them to drive. In the long term, though, cars got smaller and fuel efficiency standards rose during the 1980s (i.e., less fuel was used per kilometer or mile travelled). Expensive unconventional methods to obtain petroleum as well as renewable sources of energy also began to attract attention and investment dollars. Unsurprisingly, oil supply increased, substantially exceeding demand at times (during periods known as oil gluts). Resulting declines in petroleum prices then cut into the profits of oil producers and necessitated the closing of many costly wells. Economic, political, and military conflicts during recent years and decades have meant that price and supply remain volatile. These days, OPEC—the group that controls most of Earth’s conventional oil—is pitted in a complex battle against producers in Canada, the United States, Russia, and other countries that rely on unconventional methods. The former would like to ensure elevated and stable prices whereas the latter rely on relatively high prices to enable their continued existence even as they drive down prices by increasing supply. Put bluntly, some in OPEC have sought to flood the market with oil to lower prices and put competitors out of business. Interestingly, though, expensive unconventional wells have continued to produce petroleum when prices have decreased, in part because the industry desires to remain relevant in the long run. Of course, all types of oil producers are threatened by renewable sources of energy we will describe below.
Two final points about oil deserve some attention. First, in projecting the remaining life of oil, we should assume that its price will go up as it becomes increasingly scarce. In other words, oil will probably be around far longer than 43 – 130 years (currently, the most plausible estimates) because it will be too expensive for most people to use in any relevant quantity. Of course, in such a case it would no longer provide as much energy as it currently does, presumably due to the rising viability of other non-petroleum options, and remain on Earth indefinitely. Second and related, before we embrace the notion that 2, 4, or even 12 trillion barrels are left, a thorough examination of the consequences of extracting, refining, transporting, and burning all the remaining oil we can ultimately access is warranted (we will return to this point in Chapter 14). Informed by the data and scientific ideas presented here, we could undertake serious discussions about the future role of petroleum in meeting human demands for energy. Put into other words, although we might be able to eventually develop ways to recover every drop of oil remaining in the lithosphere, we need to properly weigh all the risks before we set out to do so.
Natural gas. The long-term viability of this fuel is difficult to predict because it depends on technology and costs of natural gas and other energy sources. With global demand at roughly 4 tcm / year and the amount in proven reserves, 200 tcm, we could conclude that the answer is 50 years[16]. The story is more nuanced, however, than this simple calculation suggests. Importantly, more than 200 tcm is likely present—possibly by a great deal—although we need to keep in mind that much of the gas assumed to be available is in reservoirs only accessible through fracking.
Coal
Coal has been prized as an energy source for centuries. It was first used to produce electricity during the mid 1880s and has been an important fuel ever since. It is made of hydrocarbons so is chemically like our other fossil fuels. As a solid, though, it is physically different and not appropriate for the same applications (e.g., coal is not as portable as oil and gas, limiting its usefulness for transportation).
Formation. Like petroleum, coal originated from organisms that lived hundreds of millions of years ago. However, in this case, the starting point was dead terrestrial plants like ferns that settled into shallow and stagnant swamps, not marine plankton sinking to the ocean floor. The remains escaped decomposition in low-oxygen environments and were transformed into an organic-rich and unconsolidated material called peat. High temperature and pressure from burial induced a reduction in moisture content as water got squeezed out, an increase in density, and a higher relative amount of organic carbon in the deposits. At depths of a few hundred meters, and after many millions of years had passed, layers (ranging from a few centimeters to several meters thick) of a rock formally recognized as coal developed (Figure 10.18).
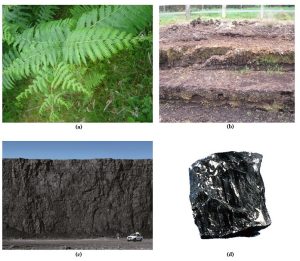
It is important to note that the term “coal” represents several subtypes, known as ranks of coal. These ranks may be viewed on a continuum, representing increasing degrees of burial, age, and energy content. Figure 10.19 summarizes some important properties of different coal ranks.
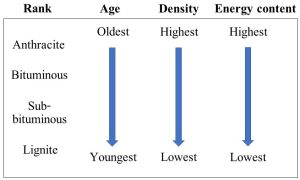
Note that energy content is directly proportional to heating value. Coal grade is another way to categorize coal, and it is a function of how much sulfur it contains and some other properties related to its usefulness for various tasks. When we consider the environmental impact of coal in an upcoming section of this chapter we will see that low-sulfur grades are preferable, if not always available.
Environmental costs. As is the case for all fossil fuels, each stage in the life cycle of coal causes adverse environmental consequences.
Extraction
Some coal is found on Earth’s surface, but much of it can only be obtained through the mining of buried material. Extraction techniques are divided into two broad categories: surface and underground mining.
Surface. When layers of coal—known as a coal seam—lie no more than about 60 meters beneath soil and rock, this approach can be used. It dominates the U.S. industry, responsible for approximately two thirds of coal extraction[17]. In short, machines remove the rocks and soils lying on top of the seam, known as overburden, and the coal is dug out and hauled away. Several specific approaches are grouped together here, including strip mining and mountaintop removal (MTR).
- Strip mining. This technique is used when coal is very close to the surface. Strip mining literally skims off long, thin swaths of overburden—strips of land—to reveal the underlying seam.
- Mountaintop removal. As the name suggests, MTR works on a larger scale than does strip mining. Here, entire mountain peaks are cleared, using explosives and related methods, and the resulting materials are deposited in nearby valleys (Figure 10.20.).
Figure 10.20. Diagram of a mountain before, left, and after mountaintop removal, right. The dotted line on the right shows the former surface of the mountain. In cross section, not to scale. Kelsey, CC BY-NC-SA.
Surface mining can cause many adverse consequences in affected areas, but just three important examples are described here. First, as land is cleared, existing ecosystems are destroyed. Consequently, the services they deliver, such as providing habitat for organisms, stabilizing topsoil, fixation and storage of carbon, and water retention, are lost as well (Chapter 5). Second, the removed overburden tends to accumulate in valleys, changing habitats and water runoff as well as spreading toxins that are the products of mining. Such areas can remain hostile to ecological succession (Chapter 5) and the return of healthy ecosystems for indefinite periods of time after mining ceases. Third, the digging of rocks and soils that have been buried for thousands or millions of years leads to a phenomenon known as acid mine drainage. Briefly, when certain sulfur-containing minerals such as pyrite are unearthed during mining, they undergo a chemical reaction that produces a very corrosive and concentrated substance known as sulfuric acid. Runoff and infiltration flowing from mines carry this acid and other toxic substances to nearby reservoirs (Figures 10.21 and 10.22).
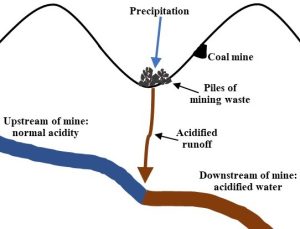
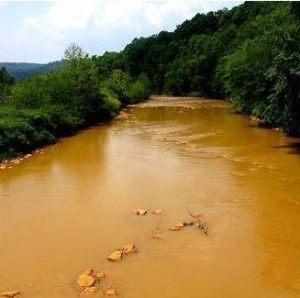
Surface mining is quite controversial, and arguments about the relationship between its costs and benefits rage. Opponents note the extensive damage caused by the practice, especially the wide-spread changes brought about by mountaintop removal; images of portions of states like West Virginia and Kentucky show some dramatic effects of strip mining and MTR (Figures 10.23.a. and 10.23.b.).
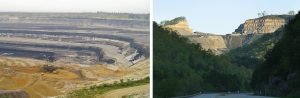
Anti-MTR people also point out the long-term loss of habitat caused by the accumulation of toxic substances in mined regions. On the other hand, proponents of the practice, including mining companies, see the situation differently. In fact, to reflect their more positive outlook, they use a different name: MTM, in which the second M refers to “mining”. They cite how surface mining allows for far more recovery of coal from a seam than does underground mining (described below) and is the less expensive of the two approaches. Risks to human life are also reduced when miners do not enter deep and dangerous shafts. Furthermore, supporters point out how federal laws require responsible parties to return former mines to their pre-mining condition. You should realize, though, that remediation of areas subjected to strip mining is extremely difficult and yields mixed results.
Underground. If coal is too deep for surface extraction to be practical, this second technique is used. In the U.S., only about one third of mining is done this way, although that number is not consistent from region to region: for example, more than three quarters of the coal taken from the Appalachian Mountains comes from underground mines[18]. These sites consist of long shafts dug from the surface down to the appropriate depth—often around 300 meters, although they can be 500 or more meters deep—and chambers that spread out laterally to follow a seam. Miners use various tools and strategies to remove coal from caverns. Rail cars and conveyor belts move the extracted rocks to the surface (Figures 10.24 and 10.25).
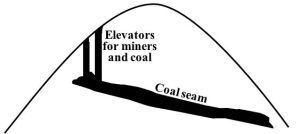
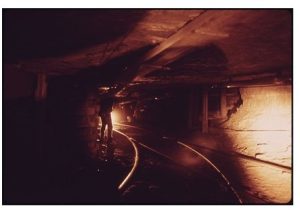
Underground differs from surface mining in several important ways. Most obviously, people must descend the hundreds of meters necessary to reach a seam and subject themselves to great danger. The risk of death from asphyxiation, exposure to poisonous gases, explosions, and mine collapses continues to be quite real to this day. Additionally, underground mining is less efficient than is surface. Since underground coal provides structural support for the mines themselves, only a fraction of it can be extracted before conditions become too hazardous to continue (the amount varies among sites).
Like surface mining, underground mining causes environmental degradation in the form of acid mine drainage (review Figures 10.21 and 10.22, above) as well as damage to existing ecosystems. The latter issue is less pronounced here because overburden is not removed, however, the roads, rails, and other infrastructure which are built to support mining are disruptive. Finally, mine fires are a serious, albeit somewhat quirky and infrequent, consequence of coal mining. Since buried coal is in a largely oxygen-free environment, it is not likely to burn while under the ground (oxygen is needed for combustion). However, once mining commences, the situation changes because shafts leading from the surface allow O2 gas into the coal seam. An explosion or other ignition source can start a fire that burns for years or decades, possibly continuing until all the coal has been consumed. Very large coal mine fires are currently burning in India, China, and Pennsylvania, U.S.A., riddling these places with sinkholes and smoking vents, and generally rendering them uninhabitable. In the last case, the town of Centralia has been essentially eliminated due to a fire burning since 1962—little more than roads and warning signs are all that remain (Figure 10.26).
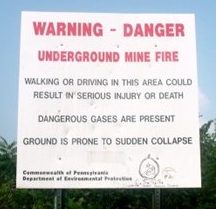
Transport
Mined coal is moved to processing plants and the places at which it is burned. As always, such transport requires energy from the combustion of fossil fuels for coal to be available to users. Of course, unlike oil and gas, it is solid rocks—so rail cars, barges, and trucks rather than pipelines are employed.
Processing
Refining of coal is not necessary to produce a useable fuel—it can be burned as soon as it is extracted. Coal is often subjected to some processing, though, to improve its quality: rocks, chemical impurities, and other materials that reduce its efficiency as an energy source can be removed before it is shipped to users. Also, it generally is pulverized before it is burned. As with all the other steps, this one is powered by the burning of fossil fuels so is associated with air pollution.
Use
The role played by coal has changed during the past century or so. Historically, energy released during its combustion was widely used to heat buildings, power the movement of trains and ships, and starting in the late 19th Century, generate electricity. During the early and middle parts of the 20th Century, coal became less and less important in transportation and the direct heating of spaces because it was replaced by various petroleum products in cars, trains, and homes. The reasons for this change include the more portable nature of liquids like gasoline and diesel as well as the relatively large amount of air pollution released when coal is burned. It continues to be heavily used in electricity generation, though (as well as in steel and cement making). Notably, it is still responsible for nearly 40% of worldwide electricity production, although its importance varies by country[19].
The combustion of coal produces the pollutants we saw for other fossil fuels. Three warrant some additional attention here, and we will return to them again in Chapter 14. First, coal burning releases more carbon dioxide per unit of energy than oil, exceeding that from gasoline by 30 – 40% (depending on the rank of coal used—recall Figure 10.19). Second, the sulfur and nitrogen oxides emitted from coal-fired power generation facilities can cause a great deal of environmental damage, particularly when high-sulfur grades are used: smog and acid precipitation are two consequences we will explore in some detail. Third, coal burning produces fly ash (light enough to rise into the atmosphere) and bottom ash (adheres to the floor and walls of a furnace), two solids not released by oil and gas combustion. These last pollutants are generally recycled and used as additives in the manufacturing of materials like cement or buried in landfills (Chapter 13).
Supply and demand. As we saw with oil and natural gas, humans have come to depend on coal. For example, in 2021, it accounted for approximately 11% of total energy supply and 22% of the electricity generation in the United States[20]. We know natural processes form this non-renewable fossil fuel far more slowly than humans use it, so we are keenly interested in the many variables influencing coal’s availability, use, and long-term prospects.
Most of the world’s coal is concentrated in just five countries
China and the United States together control almost 50% of the world’s reserves of coal, and about 30% is found in Russia, Australia, and India[21]. The rest is in South America, Asia, Africa, and Europe.
The value of coal varies
It is important to realize that coals differ in many ways and that they are not all equally valued. As we saw above, rank is one critical consideration (review Figure 10.19). Anthracite is the densest and hardest, and it is prized because of the large amount of energy released when it is burned—that is, less of it is required to power the same amount of work compared to, say, low-carbon and soft lignite. Unfortunately, anthracite is by far the least abundant (and, therefore, costliest) coal, making up only about 1% of reserves. Bituminous and sub-bituminous coals are softer coals than anthracite and have lower energy content per unit mass, however, since they are most abundant, they are most often used. A second important property is sulfur content. It is fair to say that low-sulfur coals are most sought after because they burn relatively cleanly, but as with anthracite, their supply cannot keep up with demand in many places.
The U.S. has abundant coal
The United States possesses large reserves, as well as unproven resources, of bituminous and sub-bituminous coal. It is extracted by miners across the country and has been a dominant source of energy for well over a century. More than 40% of that coal is produced in Wyoming, and most of the rest (about 30%) comes from states within the Appalachian Mountains and Illinois[22]. Worth a final note: coal from western states such as Wyoming has less sulfur in it than does that from the east.
How long will coal last?
The amount of proved worldwide reserves is 1100 billion tons of coal, and current annual demand is around 8 billion tons[23]. So, we can use the same type of simple calculation used for oil and gas to estimate that coal would last for approximately 140 years if demand remains constant. Some people are more optimistic, though (including the U.S. Energy Information Administration, U.S. EIA) projecting that supply will last another several hundred more years[24]. As with oil, different assumptions about probable supply and future demand yield vastly different predictions.
Will it be used extensively in the future?
Coal could be available for human use for many decades, if not centuries. Whether it will, or even should, be a primary energy source in the future depends on several considerations, though. First, as noted above, extraction of coal causes extensive environmental damage—the effects of surface mining, for example, are undeniable and very difficult to reverse. Second, burning of coal generates chemical and physical products that can substantially degrade the quality of air. In fact, its use as a fuel to heat American homes declined precipitously during the middle of the 20th Century in part because of the smog and other air pollution problems it caused. Since that time, laws have been enacted and measures taken to reduce the release of many of the most troubling products of coal combustion, like sulfur and fly ash, but such clean-up is quite costly. Still unsolved, however, is the problem of carbon dioxide, a major contributor to global climate change. In the U.S. alone, coal-powered electricity plants release approximately 900 metric tons of CO2 annually (or 60% of the 1600 metric tons released from all electricity generation)[25]. How to capture that gas before it enters the atmosphere is a current area of research, but practical and cost-effective solutions appear to be years away. We will revisit coal’s role in the generation of air pollution, as well as strategies used to reduce its negative impacts, in Chapter 14. Finally, the availability of other sources of energy, particularly renewables that bring about far less environmental disruption, will influence discussions of whether the costs of coal are justified by the benefits provided by its continued use.
10.4.2. Nuclear power
Now we turn from fossil fuels to explore a very different strategy to generate power. In this case, the energy of certain types of atoms is converted into electricity through means other than combustion. We begin with a brief description of the process and then will consider the environmental consequences and prospects of this non-renewable energy source. Note that not all nuclear power plants are the same, and the information provided here is intended to provide a generalized overview.
Nuclear fission releases large amounts of energy
Recall from Chapter 4 that atoms are the minuscule building blocks of everything in the Universe and that each of them is comprised of even smaller particles known as protons, electrons, and neutrons. Since these subatomic particles are held together with very strong bonds, it is quite difficult to break atoms apart. When they are split, though, during a process known as nuclear fission, an enormous amount of energy is released. Under the right circumstances, some of that energy can be captured and converted into electricity.
Unstable atoms can be split. For several reasons, most notably the fact that they are relatively easy to split, some uranium atoms (U) are well suited for nuclear power generation. In fact, certain U isotopes, referred to as U235, are so unstable that they can spontaneously break apart (the superscript indicates the number of neutrons in the atoms, which can differ for atoms of the same element). Since natural U235 is found in very low concentrations in rocks and soil, mined uranium must be extensively processed before it is fit to serve as a fuel. Several steps and specialized equipment—along with a great deal of energy—are necessary to produce a usable fuel. This product, known as enriched uranium, consists of a mixture of uranium isotopes: 2 – 4% is U235 and the rest is U238 (we will see more about this second isotope shortly). Fission (splitting) releases several products: smaller atoms (known as fragments), neutrons, ionizing radiation, and heat.
Fragments
The original, or parent atom, is converted into smaller, daughter atoms. Since the initial daughters produced are also unstable, they too break into even smaller fragments; this process, termed radioactive decay, continues through several steps until stable atoms form. Note that unstable atoms vary widely in the time required for them to become stable, ranging from fractions of seconds to billions of years. This period is quantified with a unit known as the half-life, or the time required for half of the parent atoms present in a space to undergo conversion to daughters.
Neutrons
These subatomic particles are important products of fission because after they are released they can strike other unstable atoms and initiate additional fission. Nuclear reactors rely on interactions among neutrons and fissionable atoms to operate. We will return to the important role of neutrons in power generation below.
Ionizing radiation
This is a type of invisible energy that can travel through air (or even a vacuum) and induce profound chemical changes in the objects it encounters. For example, by transforming important structures inside of cells, radiation can damage or kill organisms. Gamma radiation—a very dangerous type that can penetrate deep into both living and non-living materials—is released in nuclear fission. Bodies that emit gamma or one of several other types of radiation are said to be radioactive.
Heat
Fission of U235 also releases enormous amounts of heat, meaning that a relatively small mass of material can be used to generate a great deal of electricity (more below). Heat from fission reactions is used to produce steam.
Nuclear fission can generate power. Simply put, a nuclear reactor is a reinforced chamber into which fuel (usually the mix of U isotopes described above) is submerged in water and allowed to undergo fission. The process involves several important steps.
A neutron gets it started
Uranium atoms are placed close together, and a neutron released when one undergoes fission collides with an adjacent one. That second atom then splits and releases one or more neutrons, leading to the fission of additional atoms.
A chain reaction ensues
At first, a small number of atoms are transformed into products. As more and more are split, the number of neutrons in the reactor increases, causing more and more atoms to undergo fission (Figure 10.27). Such a process is known as a chain reaction and ought to look somewhat familiar because it resembles the many examples of positive feedback we have seen throughout this book (first encountered in Chapter 2). As the reaction grows, so does the amount of heat released into the water. Eventually, the proper temperature is reached, and steam is continuously sent from the reactor to spin a turbine.
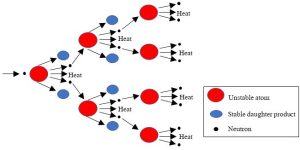
Plutonium is produced
While the U235 undergoes fission, another important process occurs inside the reactor: some of the U238 atoms present (the bulk of the material) are converted into plutonium (Pt) atoms. Atoms of one of the plutonium isotopes, Pt239, also decay and release a great deal of additional heat (which contributes to steam generation).
The chain reaction proceeds in the reactor core. A nuclear reactor consists of several essential components (Figure 10.28).
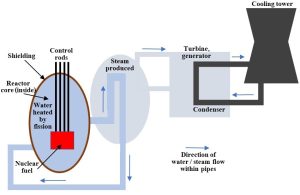
Nuclear fuel (fuel rods)
Uranium is packed into long, thin metal tubes. Several of these fuel rods are then assembled and can be lowered into a reactor.
Water
Although the details of this step vary among power plants, the heat released during fission ultimately boils water and generates steam that is directed at turbines to generate electricity. The water is maintained in a closed loop and used repeatedly, so it must be cooled and condensed after it has boiled and then cycled back into the reactor. The critical cooling step is generally accomplished using hyperboloid or hourglass-shaped cooling towers that are so often associated with nuclear power (these are large, well over 100 meters tall; Figure 10.28 shows their basic shape). A second batch of cold water taken from a nearby surface reservoir is placed close to pipes carrying hot steam from inside the reactor. Re-condensed water is then ready for another round of boiling and electricity generation.
Control rods
These metal cylinders are constructed of materials that absorb neutrons without undergoing fission and perform two important functions. First, they help keep a reaction running at a constant rate and make electricity generation as efficient as possible. Briefly, they are lowered into the reactor core once the water temperature has reached the target level and are subsequently retracted and inserted to maintain it. Second, they provide a means of reducing the risk of an accident. Without control rods, a chain reaction could proceed rapidly enough that the chamber in which it starts can no longer contain it. In other words, many atoms would simultaneously release energy and cause an explosion. For reasons that are likely obvious, operators of nuclear power plants would prefer to avoid exploding reactors. Many precautions are taken to minimize the likelihood of a runaway chain reaction, but control rods are among the most important.
Shielding
Nuclear reactors are built to prevent radiation from escaping into natural environments. Gamma radiation is the biggest concern as it can pass through solid objects (as noted above), so the core is typically constructed of thick steel and concrete. Water serves as a shield as well.
Waste is removed and stored. Two types of radioactive waste must be managed.
Low-level waste
This emits relatively little radiation. Some of the materials used to generate electricity at nuclear power plants fall into this category. Various storage strategies are used to protect organisms, soil, and water while its radioactivity is lost due to natural decay, but it generally does not pose a serious threat.
High-level waste
Although it makes up less of the total volume of waste produced at a nuclear power plant, it presents by far the bigger risk of the two types. Simply put, it consists of uranium, plutonium, and other fission products. As it contains materials that are quite radioactive (as well as very hot) this spent fuel must be handled carefully to ensure that humans and natural systems do not contact it. The first step in its management is to place fuel rods in large tanks of water for several years to allow them to cool and provide time for decay to remove some of the remaining radioactivity[26]. Then, the material is moved to dry storage. These two stages generally occur at a power plant and are intended to serve as a temporary holding strategy. Some of the waste products have very long half-lives, so they will continue to release radiation for thousands of years. Still unresolved is what to do with these dangerous materials because no permanent storage solution currently exists for the U.S. (the country that generates the most electricity through nuclear fission). We will return to this point below.
Nuclear power provides benefits and poses risks
Nuclear power has both advantages and disadvantages. Like fossil fuels and the other energy sources described in this chapter, we can use science to help us decide if the benefits of using it are worth the risks.
Advantages. There are reasons nuclear power is favored by many people as an energy source.
It releases no carbon emissions
Advocates point out that nuclear fission releases a great deal of energy without emitting carbon dioxide. Relative to fossil fuel combustion, it does not increase the concentration of CO2 in the atmosphere or directly contribute to global warming (Chapter 14).
It releases no radiation
Normally, radiation emitted by unstable atoms is contained inside the reactor by various structures.
It uses relatively small amounts of material to generate large amounts of electricity
Far more energy is concentrated in U235 than is found in coal, the largest global contributor to electricity production: just one ton of uranium can generate the same amount of electricity as 16,000 tons of coal[27].
It generates relatively little physical waste
About of 2000 tons of spent fuel is produced annually in the United States[28]. For comparison, coal combustion produces about 130,000,000 tons of ash each year[29].
Disadvantages. There are costs and risks of nuclear power and reasons to be cautious about it.
The risk of catastrophic accident is low but not zero
The many redundancies and safety features built into nuclear power plants make nuclear accidents unlikely, and it is fair to say that a great deal of power is generated without radiation leaks or other incidents. However, the potential for destructive events certainly exists, as shown by three serious accidents that have taken place in the past four decades[30]. The first was in 1979 at the Three Mile Island power plant near Harrisburg, PA (U.S.A.). In this case, the outcome was relatively minor, but some amount of radiation escaped the confines of the facility. Due to multiple errors and technical issues, one of the reactor cores underwent a partial meltdown, which is more or less what the name suggests: cooling and monitoring systems failed, allowing the core to get so hot that parts of it melted. It could have been far worse, for example, an explosion that would have exposed millions of people to high levels of radiation. As it was, public health concerns were heightened but widespread effects on the nearby population were never confirmed. Very importantly, public opinion turned decidedly against the nuclear industry, and no new facilities were opened in the U.S. during the decades following the accident (until 2023, as noted below). Furthermore, many new regulations were introduced to improve the safety of existing plants. The second occurred in 1986 in at the Chernobyl nuclear plant in Ukraine (then in the U.S.S.R.). It was a devastating accident, as an explosion destroyed parts of the power plant and allowed high levels of radiation to escape and travel thousands of kilometers throughout Europe and Asia. Around 30 people are thought to have died in the initial accident, but thousands more have been affected by chronic conditions like cancer in the years since. Today, even though the damaged reactor is encased in a containment structure, the zone within a 30-km radius of the plant is all but off limits to humans. Thirdly, the Dai-ichi Fukushima nuclear power plant of Japan was damaged by an earthquake and subsequent tsunami in 2011, leading to meltdowns of multiple reactors and the release of radiation and radioactive material into air and water. Tens of thousands of people were evacuated from affected areas, and due to continuing high radiation in many of those places, few have been allowed to return. Long-term health effects are still to be determined, but this massive accident again raised worldwide doubts about the safety of nuclear power. Some countries even vowed to abandon their usage of fission in response to the event, citing what they saw as unacceptably high risk.
We have no long-term plan for spent fuel
Arguably, this issue is the biggest problem with nuclear power and the one that is currently the most intractable. Half-lives of the different radioactive atoms in the waste range from around 30 to 24,000 years (plutonium is on that high end), meaning that the mixture of these life-threatening materials must be securely isolated for tens of thousands of years. In the United States, the need for a designated storage facility was identified decades ago, but one has not yet been built. A place in Nevada known as Yucca Mountain was extensively studied and nearly chosen as the site, but due to many scientific, technical, security, and political issues the plan has been scrapped. Other countries face similar problems. The search for a suitable solution, at a national or perhaps even international level, continues. For now, nuclear power plants produce radioactive waste and hold it in the structures we noted were only designed to be temporary solutions. Unfortunately, some of these containers are disintegrating, raising concerns about potential environmental contamination. The amount of high-level waste needing long-term storage could be reduced if spent fuel was treated to extract some of the usable isotopes and used to generate more electricity. In other words, instead of storing waste for thousands of years, the useable fraction would be reprocessed into new fuel. The U.S. has prohibited the reprocessing and reuse of plutonium from spent fuel to reduce nuclear proliferation, however (next item). Some countries using nuclear power do not adhere to such rules.
It could contribute to the proliferation of nuclear weapons
Nuclear power is sometimes referred to as a peaceful use of fission. Unfortunately, some of the same equipment used to enrich uranium for power generation can produce fuel concentrated enough for a nuclear weapon. In addition, high-level radioactive waste contains some chemicals that could be incorporated into weapons. For example, plutonium, a product of uranium decay, could be isolated from spent fuel and built into a bomb.
Cost of plant construction and decommissioning are high
Nuclear power plants are very expensive to build, operate, and ultimately shut down when they have been taken off line. The construction costs are quite difficult to estimate because they vary so much by location and often go over budget in any case; plus, since only one new plant has been opened in the U.S. (2023, in the state of Georgia) in the past few decades, the real cost of a new one is particularly hard to calculate with much certainty. A figure in the range of at least $10 to $15 billion is likely. Often overlooked by most people is the big expense that arises at the other end, that is, when a plant is shut down after its useful life has been expended (more below). Among other tasks, all the radioactive material must be handled, and the facility decontaminated. Again, it is hard to come up with a definitive figure here, but certainly hundreds of millions of dollars at a minimum is reasonable. Running a plant (labor costs, maintenance, etc.) costs money too, of course, but relatively speaking, this is a small figure.
Mining of uranium has environmental costs
As noted earlier in this section on nuclear power, uranium is obtained through mining. Although it might go without saying, a brief reminder is in order: mining is responsible for a great deal of environmental degradation. Many of the consequences of coal mining such as land clearing, ecosystem damage, soil erosion, toxic runoff, and air pollution, for example, are seen here as well.
What is the future of nuclear power?
We will end this section with some thoughts about the role nuclear power will likely play in the future and some factors affecting its long-term viability as an energy source.
Usage[31]. Nuclear power has met approximately 20% of electricity demand (9% of total energy) in the United States for most of the past 30 years and is projected to remain near those levels for the next 30. Thirty other nations rely on fission to generate electricity to different extents, such as France (where it accounts for nearly 80% of demand) and China (about 2%). Again, the U.S. generates more electricity from nuclear than any other nation—nearly double that of the second-biggest user—but nuclear accounts for a smaller percentage of its total than, say, that of France because usage in the former country is so much higher than that in the latter.
Uranium supply. Various estimates suggest the fuel supply is likely to last for another century or so, assuming neither the technology of nor demand for nuclear fission changes during that time. Now, it would be possible, under a set of unlikely circumstances, for the availability of useable nuclear fuel to be extended by many centuries if a different plant design, known as a breeder reactor, were widely used. In short, reprocessing of nuclear waste, including the plutonium we discussed above, would increase fuel supply and decrease the need for extensive management of high-level radioactive waste. Many obstacles, including very high costs to build hundreds (or more) necessary new facilities, security concerns, safety issues, and considerable political opposition to nuclear power, make such a system extremely difficult to bring to fruition. A second point about supply merits a brief comment here: although uranium mines are widely distributed, Kazakhstan, Canada, and Australia control about 70% of the world’s reserves. In other words, most countries with nuclear programs import the bulk of what they use. In the U.S., for example, domestic mining only meets about 10% of uranium demand[32].
Meeting demand as old plants are decommissioned. Nuclear plants are not designed to operate indefinitely—eventually, they must be shut down and replaced with new ones if the share of electricity generated by nuclear power is to remain constant. In the U.S., such facilities are licensed by the Nuclear Regulatory Commission (NRC) to operate for 40 years, and the current average age of the 61 facilities (99 total reactors) is 36 years[33],[34]. Now, it is common for operators to apply for (and receive) a 20-year extension to their license, and four new reactors are currently under construction. What will happen to nuclear power during the coming decades, however, is by no means certain.
Management of nuclear waste. Many people argue that nuclear fission is generally a safe, efficient, and effective way to generate electricity, but the problem of what to do with high-level radioactive waste remains unsolved. Without a permanent solution in place, nuclear power presents a continuing threat to environmental and human health. How much, and even whether, it can continue to serve as an energy source will depend in large part on how much risk people are willing to tolerate as well as how the U.S. and other countries choose to manage their waste in the future. We will return to this and related questions when we explore the science of risk assessment in Chapter 15.
A role in efforts to combat climate change? As noted here already, under normal circumstances, nuclear power plants emit no carbon emissions. We will learn a lot more about the effect of CO2 on climate in Chapter 14, but for now let us just say that meeting growing worldwide demand for electricity through fossil fuel combustion will be highly problematic. Some argue that nuclear fission provides an environmentally friendly way to provide power without air pollution and should, therefore, be expanded. Work to design safer and cleaner reactors that generate less-risky waste is ongoing, but whether it produces viable alternatives to other sources of energy remains to be seen.
Part III: RENEWABLE ENERGY SOURCES
10.5. RENEWABLES OFFER ALTERNATIVES
Renewable energy sources—sometimes called alternative energy sources—have become increasingly relevant during the past few decades for two reasons. First, on-going volatility in the price of petroleum and worries about the long-term supplies of fossil fuels have driven many to explore options that are, at least in principle, free and inexhaustible. Second, extracting, processing, and using non-renewables lead to substantive environmental degradation, including climate change (Chapter 14). Although renewables are by no means without environmental cost, it is fair to say they are generally more benign than non-renewable sources. Here, we will examine several renewable energy sources, briefly considering how they are harnessed to generate power, their advantages and challenges, and their potential to supply energy in the future.
10.5.1. Direct solar
The sun provides power for nearly all the processes active on Earth, including photosynthesis, wind, and the cycling of water. These and other indirect manifestations of solar energy are clearly vital to human survival, as described in previous chapters. An understanding that the sun also can serve as a direct energy source developed relatively recently, although it is hardly a new idea: people have exploited the sun’s heat to raise the temperature of homes, dry objects, and even cook food, for millennia. During modern times, we have developed additional uses for direct solar, namely, to heat water and interior spaces as well as produce electricity.
Ways to use solar energy directly
Heating. The sun’s energy can be harnessed to raise the temperature of water or air through one of two mechanisms. In the first, passive heating, objects are exposed to sun and their temperature is increased without the aid of any moving parts or distribution systems. For example, windows can be installed in the south-facing side of a building to maximize natural heating of an enclosed space (Figure 10.29).
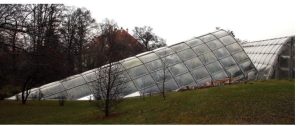
The second is known as active heating. Here, a substance with a high capacity to absorb and hold heat (a fluid of some type) is exposed to sunlight, and a mechanical system then distributes the heat. The pumping of sun-heated water throughout pipes in the walls of a home is just one application of this approach. Solar-energy-driven domestic hot water heaters are also a very common use of active heating.
Electricity generation. Electricity can be produced from solar energy using two distinct approaches.
Photovoltaic cells (PV cells)
These are made of specialized materials that absorb solar energy in such a way that electrons are induced to move (i.e., electricity is generated). Individual cells are generally small and flat, a few centimeters square and less than one millimeter thick, but many of them can be arranged together on the surfaces of solar panels (Figure 10.30).
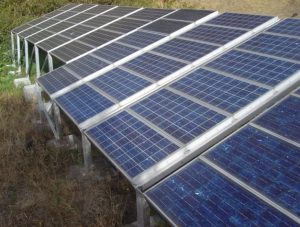
The size of panels depends on how much electricity is needed, so just two or so centimeters will suffice to run a calculator, but several panels that each are a meter or more are required to provide enough power for an entire house. Hundreds of panels arranged on a rooftop or in an open field could generate larger amounts of electricity, enough to supply many homes, businesses, or communities with needed energy.
Thermal power generation facilities
The idea is deceptively simple: heat from the sun is used to generate steam which then spins turbines. The large scale of these operations presents design challenges that have been approached in different ways. In any case, a great deal of sunlight must be directed at a vessel of water for this to be an effective system. For example, moving mirrors can concentrate the sun onto a relatively small area, raising the temperature of a fluid to nearly 500 ºC (around 1400 ºF).
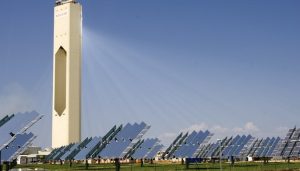
A small number of thermal power generation plants are operational, and research continues into ways to improve them. Figure 10.31 shows a solar power tower, one such facility.
Advantages and challenges of solar
Interest in solar energy has increased steadily during the past few decades for a simple reason: it holds great potential as an energy source. However, some challenges continue to limit its widespread use and ability to replace non-renewables such as coal. Here we take a look at both the ups and downs of solar power.
Advantages. The list of reasons to embrace solar power consists of economic, environmental, cultural, and security-related arguments.
Its supply far exceeds demand
The enormous amount of energy moving from the sun to the Earth more than meets our needs. One useful way to visualize the quantity is suggested by the U.S. Department of Energy (DOE): enough energy hits the Earth in 90 minutes to meet human demands for a year[35].
The energy itself is free
Solar energy is readily available and not controlled by any one country or group. To be sure, some places on Earth receive more than others, but it is accessible to anyone with the proper equipment to harness it.
Its environmental impact is low
As we will see below, solar energy is associated with some amount of risk to natural systems. Relative to fossil fuels and nuclear power, however, the effects of solar are small. Importantly, solar power is classified as a non-carbon energy source, and, as such, it does not release carbon dioxide (or any other air pollutants, for that matter) into the atmosphere.
It is renewable
Solar power is listed in this section because it fits our definition of renewable energy. It is inexhaustible in any practical way, potentially providing energy to humans in perpetuity (or as long as we are likely to need it, i.e., it will not run out for about 5 billion years).
Challenges. If solar is so great, why doesn’t it make up the bulk of our energy supply? Some of the answers can be found on the following list.
It is dispersed
This problem is linked to the first item on the list of advantages. Yes, supply exceeds demand, but the energy is spread out over the entire planet. In other words, each square meter of the surface receives a small percentage of all incoming sunlight. Panels must therefore be large if they are to collect enough solar energy to generate relevant amounts of electricity.
It is variable
As is quite familiar, the amount of sun striking a portion of the Earth is not constant. For example, the sun sets in the evening, cloud cover changes, days are shorter in winter, and equatorial regions receive more direct sunlight than do those at high latitudes. Put another way, solar energy is intermittent and variable. These and other factors mean that the amount of electricity generated from solar power will differ in both space (i.e., location), and time (i.e., season, month, and hour). It is simply a reality that some areas are better situated for solar power than are others. For example, it is more efficient and realistic in southwestern portions of the United States than it is in northern Canada.
There are technological obstacles
The first two problems on this list, the dispersed and variable nature of solar energy, are made even more challenging by the state of the currently available technology. Even though we have clearly learned to effectively capture energy from the sun and convert it into heat and electricity, some improvements would allow solar power to make a more substantial contribution to our energy supply. For example, photovoltaic cells are inefficient, converting only about 20% of received solar energy into electricity (worth noting, though, is that efficiency has been steadily improving during recent years). Furthermore, better batteries or other storage solutions are needed to increase the viability and relevance of solar power.
It is costly to harness
We noted above that the energy itself is free and readily available. The technology required to convert sunlight into electricity requires a financial investment, however. Yes, it has gotten steadily cheaper during the past few decades—and is competitive with or even less expensive than conventional energy sources in a few places—until recently the cost of solar has been quite a bit higher than that of fossil fuels and only affordable by a small number of people. Centralization is an important key to the viability of solar: large power generation plants that use photovoltaic (PV) systems can provide the same quantity of electricity for far less money than can an equivalent combination of small PV systems installed on individual homes.
Solar brings its own environmental disruptions
Solar power has a relatively small effect on natural systems, but two important concerns are worth noting. First, the chemicals in batteries and photovoltaic cells are toxic and therefore pose a risk to humans and other organisms if not properly managed.
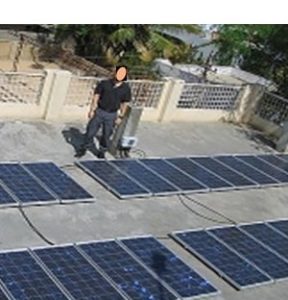
Second, given the need for large panels noted above, solar arrays cover a lot of surface area. Land clearing can affect ecosystems and increase rates of erosion (we have seen as much in Chapter 9 and earlier in this chapter) but is not inevitable if panels are placed on existing buildings and other structures instead of on open ground (Figure 10.32).
How much energy could we get from the sun?
Stooping to the use of a somewhat painful pun, the future of solar could be very bright—if the challenges enumerated above are met. As long as coal and natural gas remain cheap compared to solar, though, the former sources will continue to thrive. Certainly, concerns about air pollution and other environmental degradation associated with fossil fuels will drive some shift toward solar, but cost is likely to exert more influence over energy choices made by individuals and governments. What if we ignore costs for the moment? How much of our demand for energy could realistically be met by the sun? If you consider that only about 3.7% of worldwide electricity currently comes from the sun[36], you might not be surprised to read that a lot of untapped potential is still out there. According to estimates described by the United States Department of Energy (DOE), if fully exploited, rooftop PV alone could meet 39% of U.S. energy demand[37]. The addition of large PV power generation plants would increase that number considerably, optimistically up to 90% under, admittedly, extraordinary circumstances. Many governmental and private organizations have weighed in on the question, and, depending on the assumptions they make, the amount of electricity likely to come from the sun will range between 3 and 30% by the year 2040. Put another way, the gap between potential and actual amount of energy supplied by solar power will probably continue to be large, but just how big the difference will be is very hard to predict with certainty.
10.5.2. Wind
Due to many factors, including differential heating by the sun, the temperature of Earth’s atmosphere is not uniform. The resulting pockets of relatively warm and cool air move, and wind is generated. Humans learned thousands of years ago that wind can be harnessed to do work, including powering sailing ships and pumping water. Wind was first exploited to generate electricity on a small scale in the late 1800s, became more efficient and viable in the 1970s, and expanded in its importance during the past three decades. Today it is widespread, relatively mainstream, and likely to supply an increasing amount of electricity in the future.
Converting wind to electricity
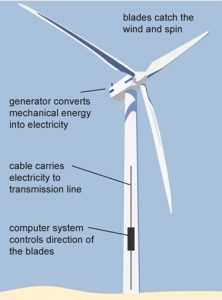
Turbines spin. We saw earlier that electricity can be produced when steam turbines are connected to a generator. Wind energy is similar: structures known as wind turbines are turned by moving air, and that kinetic energy is converted into electricity (Figure 10.33). There are some differences, such as wind turbines do not spin at a constant rate, but many of the basic principles are the same.
Scale varies. The size and number of wind turbines constructed in an area affects the amount of energy captured. For a single house, one small turbine is generally sufficient, but larger numbers of turbines, each of which could be 60 meters tall and possess 30-meter-long blades, can be clustered together in a centralized wind farm (Figure 10.34) to produce enough electricity to meet demand for communities and cities.
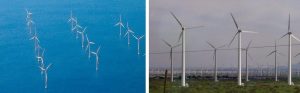
There are many wind farms in operation. For example, the Horse Hollow Wind Energy Center in Texas (U.S.A.), has over 400 turbines on 47,000 acres of land and can supply enough electricity for about 300,000 homes[38]. Of course the amount of electricity produced depends on how much wind is blowing at a given moment, a concern we will revisit shortly.
Advantages and challenges of wind energy
We have improved our ability to harness the wind, and it has become a practical and affordable source of energy. At the same time, though, some obstacles to wider usage persist.
Advantages. Many of the items on the list for solar are here as well: the energy is free and accessible, produces no chemical pollution and otherwise has a low environmental impact. Furthermore, it is abundant, although its supply does not exceed our demand for energy (more below) and, of course, it is all but inexhaustible.
Challenges. Wind has some problems in common with solar.
Relatively high costs historically
The required technology was expensive for decades, although costs are now about the same as those for coal. Past high prices delayed its growth, and only in recent decades has it become attractive as an alternative to fossil fuels.
It is variable and intermittent
Some areas simply receive more wind than others, and it is not constant with time, weather, or season.
Some environmental degradation
A great deal of space is needed for a wind farm. As we saw with solar, though, turbines could be placed in areas that already have been cleared (e.g., agricultural fields). In addition, spinning turbine blades in terrestrial farms could harm birds and bats, and marine systems can be disrupted by off-shore farms. Audible warning signals and appropriate location choices can minimize these problems.
Aesthetic degradation by windmills
The aesthetic degradation brought about by wind farms has raised concerns about and opposition to the construction of electricity plants powered by wind. On the other hand, some point out that a coal-powered plant, with its smokestacks and other features, is at least as objectionable.
How much energy could we get from wind? How much will we get?
The amount of energy wind contributes to the U.S. electricity supply has increased from 1 to over 9% since 1990, and it currently meets about 6.5% of total worldwide demand[39]. China, the European Union, the United States, and Germany have the most installed wind power, but more than 100 countries use this renewable source to varying degrees[40] Clearly, it is still a minor piece of the overall energy picture, but interest has grown as prices of wind turbines have fallen and concerns about fossil fuels have increased. Wind’s role in electricity generation may increase, but there is not agreement on just how important it will become. For instance, the International Energy Agency suggests wind could generate nearly all of our electricity if offshore farms (i.e., turbines are located in the ocean, not on the mainland) were fully utilized[41], whereas a report by the U.S. EIA is more modest in its prediction that it will account for about 31% of our supply by the year 2050[42]. As with solar, the role of wind during the next several decades will depend on the cost and reliability of fossil fuels, public support, as well as trends in air pollution expectations and laws (more below).
10.5.3. Hydro power
Put simply, hydro power refers to various ways to capture the energy associated with flowing or falling water, such as that in a river channel, waterfall, or an ocean. The use of the energy of moving water is not a new idea; like wind, humans have been using hydro power for millennia in the operation of mills and other machinery. Hydro power was first used to produce electricity in the late 1880s and was the dominant renewable energy source for decades. In fact, until quite recently, it contributed more energy than solar, wind, and the others on this list combined. It was overtaken by wind recently and still lags behind fossil fuels and nuclear, though, despite its potential.
How the energy of moving water becomes electricity
The story should be familiar by now: turbines are spun, this time by water moving from high to low elevation (or by waves or changing tides), and electricity is generated as we described earlier in this chapter. The details of how the process is accomplished vary from place to place, with one of two general strategies used most often in hydroelectric power plants. In the first, turbines are placed within an unaltered body of moving water and spun by the natural current. In the second, a dam is built across the path of a river. Here, a reservoir of water is formed upstream and water is released through relatively narrow pipes in which turbines are installed. This strategy allows for the flow rate—and resultant electricity production—to be controlled and constant (Figure 10.35).
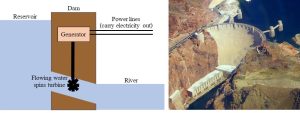
Advantages and challenges of hydro power
Advantages. As with solar and wind, there are many compelling reasons to use the energy of water to generate electricity.
The energy is free and accessible
Water flows naturally in response to gravity, and its energy can be harnessed without the need for mining or other complex extraction techniques.
There is no chemical pollution of water
Hydro power uses moving water and does not introduce any toxic substances into reservoirs.
It has a low environmental impact
Compared to the many consequences of fossil fuels and nuclear, hydro power is relatively clean, requiring no extraction, transport, refining, combustion, or extensive waste management.
It uses proven and cost-effective technology
We have been generating electricity with hydropower for over a century and have developed practical and efficient ways to do it. In fact, when total costs are considered (construction, maintenance, fuel, and labor), hydro power is considerably cheaper than nuclear and about equal to fossil fuels.
It is consistent
Unlike solar and wind, hydro power can be captured and released at a constant rate. Among other benefits of this trait is the way it can be used in conjunction with intermittent renewable sources like solar and wind to provide uninterrupted power.
It is renewable
Although not as abundant as solar, its supply is inexhaustible under any likely scenarios.
Challenges. As you likely expect, there are some down sides of hydro power.
An initial financial investment is necessary
This problem certainly is not unique to hydro power—construction costs for a nuclear plant, for example, are far higher—but this challenge cannot be ignored for any renewable on our list.
Its feasibility varies
Simply put, hydro power is not a good option everywhere because it depends on the presence of appropriate topography (remember, downhill flow is an inherent part of the process), precipitation, and space. In the case of ocean-related power, proximity to a coastline is clearly required.
It can disrupt existing ecosystems
Dam construction brings big changes, including the flooding of large terrestrial areas (review Box 4.4, including Figure 4.17, for many important consequences of dams). Often overlooked is how the creation of a large, relatively stagnant reservoir on the upstream side of a dam can increase anaerobic decomposition and the release of methane. This is no trivial matter: levels of methane in the atmosphere are directly linked to Earth’s temperature and affect climate (Chapter 14).
Dams can displace people
Flooding pushes many terrestrial organisms out of an area and fundamentally changes the character of existing ecosystems. It can also cause a great deal of human suffering as people are forced to evacuate their homes before they are permanently inundated with water. For example, the Three Gorges Dam in China (built during the 1990s and early 2000s), created the largest hydro power facility in the world by flooding about 1000 square kilometers of land[43]. Although the project provides vast amounts of electricity, it is controversial due to the ecological damage it caused and because it forced over one million people to relocate.
What is the future of hydro power?
Both the present and past suggest a long-term role for hydro power going forward. Currently, it contributes about 6% of the electricity used in the United States, second among renewables to wind (which, as we learned, is 9.2%), but it accounted for nearly all of renewable electricity from 1950 to the mid 1990s[44]. Some countries generate a much larger fraction of their electricity than the U.S., if a lower total amount overall, with hydro power (e.g., Norway meets over half of its demand this way). As of 2020, it was responsible for about 17% of worldwide electricity production[45].
The extent to which hydro power will meet energy demand in coming years depends on how much untapped capacity can be exploited by both improving existing facilities and constructing new ones. The story is an interesting and complicated one, in part because many dams were built for purposes other than power generation (flood control, etc.). In other words, the environmental and financial costs associated with them have already been borne, yet they produce no electricity. Although it is difficult to predict an exact amount with certainty, hydro power has the potential to contribute substantially more electricity than it does currently. Conservative interpretations of available data suggest 15% of U.S. electricity could come from this renewable resource, with more optimistic estimates ranging up to about 30%.
10.5.4. Biomass
The general term biomass refers to living material in plants, animals, and microorganisms and is often used by ecologists when describing structure and function in ecosystems (Chapter 5). It has a related meaning in our current discussion of energy: biomass fuels, often called biofuels, are produced or derived from present-day organisms (as opposed to ancient ones in the fossil fuels seen earlier). Of the many products that could be included in this discussion, we will take a brief look at just three: liquid ethanol, wood, and biogas. Before we proceed, a small caveat is in order. Many people categorize these energy sources as renewable because they are ultimately powered by the sun; indeed, they are also grouped like that by the author of your textbook (if reluctantly!). Arguably, though, because they are not necessarily replenished as readily and completely as are solar, wind, and hydro power, and depend on things like fertile soil for their production, this classification may not be appropriate. We will see more about this issue below.
Ethanol (or ethyl alcohol)
Ethanol is produced by fermentation, a process whereby crops such as sugar, wheat, or corn are metabolized by a type of yeast, one of the microorganisms we encountered in Chapter 3. It is familiar to many people as “alcohol”, the intoxicating ingredient in beer, wine, and liquor. In the current context, though, we are interested in this substance as an energy source. To summarize its value, ethanol is a relatively clean-burning liquid added to fuel to reduce air pollution and extend our petroleum supply.
Ethanol use is higher in some countries than others, but it still only makes up a small fraction of the global energy supply. In the early 2000s, it appeared poised to grow substantially and become far more important, perhaps even replacing gasoline completely. So, what happened? The answer is complex, consisting of many parts. One of many issues is related to reduced fuel economy: since its combustion releases less energy than does that of gasoline, engine efficiency declines as ethanol content in fuel rises. A more fundamental problem is the fact that ethanol is an agricultural product. Extensive production of corn and other plants causes the many adverse consequences of farming we explored in Chapter 9 (some, like corn, are more damaging than sugar and others). Because it depends on conventional agricultural practices that are unsustainable (loss of fertile soil being among the more notable), it is arguably unfair to call ethanol renewable. Furthermore, some people hold that photosynthesis completely offsets the carbon dioxide ethanol combustion adds to the atmosphere, but such an assertion ignores the many ways farming in general also leads to the production of this important greenhouse gas (e.g., fossil fuel use and stimulation of aerobic decomposition—again, review the link to Chapter 9). Finally, the opportunity-cost associated with using agricultural fields and resources to grow fuel instead of crops deserves some attention. Since Earth is closed with respect to materials, including arable land, whether we can expand biofuel production and still meet ever-increasing demand for food is not altogether clear.
Wood
If not strictly a biofuel like ethanol, wood certainly is an energy source derived from biomass. People have burned wood for heat for many thousands of years, and it continues to be the primary fuel source throughout the developing world. Even today, a fraction of home heating in the developed world is accomplished with various types of wood stoves. Trees present many advantages, including their accessibility and versatility Moreover, relative to fossil fuels, wood is readily replenished (requiring decades rather than millions of years). However, the harvesting and combustion of wood brings with them several adverse consequences (briefly presented here—we will return to forestry and deforestation in Chapter 12). First, the growth of relevant numbers of trees requires hundreds of thousands of acres of space, water, healthy soil, and nutrients in natural ecosystems or tree farms. Clearly, land and other resources dedicated to tree farming cannot be used for other purposes such as housing, industry, or food production. Second, widespread removal of trees can cause substantial damage to forest ecosystems (Chapter 12), increase rates of soil erosion (Chapter 9), and affect the hydrologic cycle by changing runoff and infiltration. Third, wood burning converts organic carbon stored in trees to inorganic carbon dioxide. In other words, instead of acting as a reservoir for the storage of C, forests become a source of CO2 released into the atmosphere (we will see more about this point in Chapter 14). Keep in mind that many other air pollutants are released by combustion, including gases that are toxic to humans and other animals. Finally, although wood can, in principle, be grown sustainably, trees are often removed faster than they are replaced. Yes, its energy source is the sun, but whether in practice wood is truly renewable is debatable.
Biogas[46]
This is a general term that includes methane-containing products from anaerobic decomposition (Chapter 4) of organic carbon in animal waste (Chapter 9), sewage (Chapter 11), and landfills (Chapter 13). In these and other cases, methane must be separated and concentrated from a gas mixture. It can then be transported and burned to generate electricity just like the methane described in the discussion of petroleum, above. It currently makes up a small fraction of supply, but it has the potential to grow in coming years. Again, we can raise the question: is biogas really renewable? When we consider all that is required to produce it, including farming, we should be prepared to provide a nuanced answer!
10.6. PRIMARY VS. SECONDARY ENERGY SOURCES
Petroleum, radioactive materials, sunlight, wind, hydro power, and biofuels are all known as primary energy sources because they can be processed through combustion or other means to release energy for transportation, electricity production, or temperature control. A secondary energy source, on the other hand, can be viewed as a sort of go-between that carries energy from a primary source to a place in which it can be used. Electricity is a good example of a secondary energy source because it is derived from something like the combustion of coal or the spinning of a turbine by moving water. The electricity itself is not a source of energy, rather it is a readily used form of energy that can be distributed via power lines to homes and other buildings.
Another important secondary energy source is hydrogen. This gas can store large amounts of energy, is quite portable, and can provide extremely clean power to cars and other vehicles. It also lends itself to easy transport and distribution via existing infrastructure such as pipelines. However, it has some serious limitations. Most importantly, H2 gas is difficult to obtain in the quantities needed. Certainly a vast number of hydrogen atoms are present on Earth, but nearly all of them are bound up in either H2O or CH4 and must be separated from these larger molecules before they can be used. Several strategies are available, but the most widely used and feasible ones require a large energy input—that is, a great deal of primary fuel must be expended to produce this secondary fuel. For example, if an electric current is passed through water, the bonds between the hydrogens and oxygens will break; this is done easily enough, except you should note it requires electricity. As we know, renewable or non-renewable energy sources which bring on varying amounts of environmental degradation can be used, but most of our electricity comes from fossil fuels. In short, the feasibility and wisdom of using hydrogen will depend on the primary sources used to produce it.
Part IV: CLOSING WORDS AND FUTURE TRENDS
10.7. THE STATUS QUO
10.7.1. Many sources combine to make up energy supply
No single primary source currently meets all our energy demand. In fact, it is reasonable to visualize our supply as if it were a puzzle composed of several pieces. Because some of the fuels play larger roles than others, though, the sizes of those pieces are unequal. If we combine oil, natural gas, and coal into one group and renewables into another, fossil fuels clearly contribute the vast amount of energy to the U.S. and most of the industrialized world, accounting for about 80% of the total energy supply for most of the past few decades (Figure 10.36).
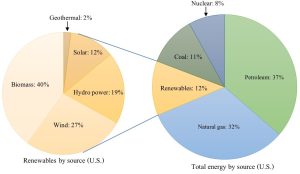
10.7.2. Renewables play a relatively small role
As we have learned, solar, wind, and hydro power offer many advantages—including the fact that they will not run out in any relevant way—yet they continue to meet less of our demand than their potential suggests. You might wonder: why is this transition taking so long? The short answer is that several factors, some of which are described below, have put up various amounts of resistance through the years.
Existing infrastructure
The Industrial Revolution was powered by, and modern societies still largely run on, fossil fuels. These non-renewable fuels are simply an integral part of day-to-day existence: transportation, electricity, heating, food production, manufacturing of plastics, cosmetics, drugs, and building materials, and many other processes are enabled by oil, coal, and natural gas. To reduce our reliance on fossil fuels, many modifications to the way we live clearly would be required. Think, for example, what a transition away from gasoline-powered cars would involve. We would need new mechanisms for fuel transport, battery charging, refueling, and service. Mass transit would also likely need to take on an expanded role. A related issue: people often find it difficult to accept any kind of changes, particularly those that threaten long-standing and entrenched conventions.
Lack of government support
Historically, the U.S. government provided far more financial support to the fossil fuel industry than to various entities working on renewable energy. Thus, private petroleum companies, which are usually very profitable in any case, have enjoyed tax breaks for oil exploration and other activities whereas research that could lower costs and improve performance of photovoltaic cells and batteries received comparatively little funding. Recent developments may lead to changes in the balance, however. The Inflation Reduction Act, passed in the U.S. 2022, includes large increases in funding for solar, wind, and other technologies defined as “clean energy”, and China, the European Union, and countries in other regions have made similar investments[47].
Perceived economic risks
Opponents of an intentional transition away from non-renewable fuels to renewable ones often cite a fear of economic hardship as a concern. Although changes certainly would occur, evidence suggests that the relative growth of solar, wind, and related energy sources would not necessarily damage our economy, rather, it would likely lead to new opportunities, industries, long-term jobs, and resilience against inflation.
10.8. AN ALTERATIVE FUTURE?
Where do we go from here? Non-renewables will not be able to meet demand indefinitely, and their continued use threatens natural ecosystems along with human well being. Could renewables take on a larger share? Various models predict that the contributions they make to the world’s total energy supply will rise from their current level of 12% to around 27% by 2050 (for electricity alone the number is expected to increase from 28% to 56% during the same period[48]). Could we do better? Scrutinizing the potential of solar, wind, and hydro power to provide power (data we explored in our earlier discussions) we see that far more than half of our total energy could come from renewable energy sources. That is, 27% could be the worst case, not the best.
Among the many benefits of a future powered by renewables: the multiple costs of dependence on fossil fuels would be substantially diminished. The proposed shift would not be simple, though. Numerous changes to infrastructure and expectations, including the three described below, would be required.
10.8.1. Increased production of secondary sources from renewable primary sources
Petroleum is a highly practical fuel for transportation because it is energy rich and portable, and any replacement would need to possess the same characteristics. Both hydrogen and electricity—two commonly used secondary sources noted in section 10.6—could fill this role if they were generated in centralized locations with abundant sun, wind, or flowing water and then distributed from there to users. See Box 10.4 for a related discussion.
Box 10.4. More electric cars?
Electric cars have become more and more common, but they still make up a small proportion of vehicles on the road. Can we increase their use? The answer depends on cost and convenience, as is so often the case. Prices of many electric vehicles have started to approach those powered by gasoline, but the limitations of batteries persist to some degree. Frankly, people are hesitant to invest in a car that has an insufficient range and is difficult to recharge. The first issue is linked to our discussion of the need for better battery technology, and the situation has slowly improved during the past several years. Re-powering electric cars remains a hurdle, particularly in the United States, but the number of public recharging stations is on the rise. Unfortunately, the time required to get back on the road during a lengthy trip can be prohibitively long, though.
At this point, a second, very important, question about electric cars must be asked: should we increase their use? Here, the answer depends in large part on the energy source for electricity generation. Fossil fuels could certainly do the trick, but using them would hardly alleviate the problems associated with these non-renewable energy sources. Urban smog would diminish due to less petroleum combustion in cities, but coal or natural-gas burning would increase elsewhere as more electricity is needed. It is true that the amount of air pollution, notably CO2, is lowered slightly overall by a conversion away from gasoline-powered vehicles, but it would likely worsen in the long run as demand for electric cars rises worldwide. In other words, if you plan to stick with fossil fuels as your primary energy source, electric vehicles provide minimal benefits. If, however, solar, wind, and hydro power are the sources used to generate electricity, a transition to electric cars would solve several problems.
10.8.2. Improvements and expansion
Technological improvements
We have already discussed many of the obstacles limiting the feasibility of renewables, but two merit a brief reiteration here. The feasibility of renewable sources to meet more of our energy demands would be substantially enhanced with increased efficiency and affordability. Research has led to improvements in recent years, and the work continues. In addition, variable sources like solar would be more attractive and portable with enhanced battery technology, a need we noted earlier.
Biofuel production
Production of fuels from biomass could be an important piece of the energy puzzle, but as we know, it is limited in large part by land use and other issues related to soil-based agriculture. Ethanol, for example, is not particularly practical if it is derived from a resource-intensive crop such as corn. However, when it is produced from sugar, other plants, or even portions of plants that are otherwise of no use as food, it could make a substantive contribution to our energy supply. Research into biofuels is ongoing, and includes work to produce fuels from giant kelp, large algae that do not require soil because they are grown in the ocean.
10.8.3. Living off the grid
Most of the developed world is designed around centralized power-generation plants that supply electricity to communities of people. The network of generators and power lines is assembled into the so-called grid we mentioned at the beginning of this chapter and is managed on a large scale to maximize efficiency and electricity availability for all. Individual users pay separate bills to the power company according to how much electricity they have used. In recent years, renewable energy sources have enabled more and more people to use solar or wind to generate electricity on a small scale—on their own property or rooftop, for example. Some structures are not connected to the community grid at all whereas others use electricity from a power plant as a back up only. This emerging type of infrastructure is creating an important new trend because of the freedom it gives people to choose how they obtain power. Moreover, it allows regions within the developing world that would otherwise be remote from a large power plant and its grid to obtain electricity through other means. Local access to power enables improvements in standards of living without the cost and environmental degradation associated with the construction and use of coal or nuclear plants.
THE CHAPTER ESSENCE IN BRIEF [49]
Demand for energy to power technology is both high and increasing. Non-renewable fossil fuels have dominated supply since the start of the Industrial Revolution and continue to do so despite the substantial environmental degradation they cause and the availability of many relatively clean, renewable energy sources. A move away from the status quo has started, yet change has been slow for multiple reasons.
Think about it some more…[50]
Besides meeting our basic biological needs, what do humans do with energy?
Why is oil classified as a non-renewable source of energy if it is formed by natural processes? Why is the sun classified as a renewable source of energy if it is not being replaced?
Imagine we discover a previously unknown oil reserve, one that could easily meet our demand for energy for 500 years. Would you embrace it? Would our problems be solved?
What variables would you consider in a risk-benefit analysis (Chapter 1) of nuclear fission and coal combustion as energy sources? Which do you think would come out on top: risk or benefit?
Some people consider ethanol to be a renewable source of energy. Given what is required to produce it, do you agree with this characterization?
Why do fossil fuels continue to meet a large majority of our energy demands when alternatives such as solar, wind, and hydropower could (mostly) take over?
- National Commission on the BP Deepwater Horizon oil spill and offshore drilling, 2011, Deep Water: the gulf oil disaster and the future of offshore drilling. Public domain. https://www.gpo.gov/fdsys/pkg/GPO-OILCOMMISSION/pdf/GPO-OILCOMMISSION.pdf ↵
- U.S. Energy Information Administration, 2022 ↵
- States National Oceanographic and Aeronautics Administration, Office of Response and Restoration, 2017. http://response.restoration.noaa.gov/oil-and-chemical-spills/oil-spills/largest-oil-spills-affecting-us-waters-1969.html ↵
- U.S. Energy Information Administration, 2022 ↵
- U.S. Energy Information Administration, 2022 ↵
- U.S. Energy Information Administration, 2022 ↵
- U.S. Energy Information Administration, 2022 ↵
- opec.org ↵
- U.S. Energy Information Administration, 2022 ↵
- U.S. Energy Information Administration, 2022 ↵
- U.S. Energy Information Administration, 2022 ↵
- U.S. Energy Information Administration, 2022 ↵
- U.S. Energy Information Administration, 2022 ↵
- Gas Market Report, Q3-2022. International Energy Agency. www.iea.org/reports/gas-market-report-q3-2022 ↵
- for example, see opec.org ↵
- U.S. Energy Information Administration, 2022 ↵
- U.S. Energy Information Administration, 2022 ↵
- U.S. Energy Information Administration, 2022 ↵
- International Energy Agency. iea.org ↵
- U.S. Energy Information Administration, 2022 ↵
- U.S. Energy Information Administration, 2022 ↵
- U.S. Energy Information Administration, 2022 ↵
- World Coal Association. 2022. What is coal and where is it found? worldcoal.org ↵
- U.S. Energy Information Administration, 2022 ↵
- U.S. Energy Information Administration, 2022 ↵
- You should realize that the only known remedy for radiation is time ↵
- Argonne National Laboratory, U.S. Department of Energy Office of Environmental Management, 2017, Depleted UF6 guide. http://web.ead.anl.gov/uranium/guide/facts/ ↵
- Argonne National Laboratory, U.S. Department of Energy Office of Environmental Management, 2017, Depleted UF6 guide. http://web.ead.anl.gov/uranium/guide/facts/ ↵
- U.S. Environmental Protection Agency, 2017, Coal ash basics. https://www.epa.gov/coalash/coal-ash-basics ↵
- U.S. Nuclear Regulatory Commission, 2017. https://www.nrc.gov/ ↵
- U.S. Energy Information Administration, 2022 ↵
- U.S. Energy Information Administration, 2022 ↵
- U.S. Energy Information Administration, 2022 ↵
- Other countries have their own strategies for oversight and management, and the International Atomic Energy Agency works to promote the safe and peaceful use of nuclear power worldwide. ↵
- U.S. Department of Energy, 2022. energy.gov ↵
- Piotr Bojek. 2022. Solar PV. The International Energy Administration. https://www.iea.org/reports/solar-pv ↵
- Gagnon, P., Margolis, R., Melius, J., Phillips, C., Elmore, R., 2016, Rooftop solar photovoltaic technical potential in the United States: a detailed assessment. National Renewable Energy Laboratory, U.S. Department of Energy, Technical Report NREL/TP-6A20-65298 ↵
- U.S. Energy Information Administration, 2022 ↵
- Hannah Ritchie, Max Roser and Pablo Rosado. 2022. "Energy". Published online at OurWorldInData.org. Retrieved from: 'https://ourworldindata.org/energy' ↵
- U.S. Energy Information Administration, 2022 ↵
- Wind. 2022. International Energy Agency. www.iea.org/fuels-and-technologies/wind ↵
- U.S. Energy Information Administration, 2022 ↵
- https://www.usgs.gov/special-topics/water-science-school/science/three-gorges-dam-worlds-largest-hydroelectric-plant ↵
- U.S. Energy Information Administration, 2022 ↵
- International Energy Agency. 2021. Hydropower Special Market Report. www.iea.org/reports/hydropower-special-market-report ↵
- For further reading on biogas, consult www.iea.org/reports/outlook-for-biogas-and-biomethane-prospects-for-organic-growth/an-introduction-to-biogas-and-biomethane ↵
- The International Energy Administration. 2022. Global Energy Review. iea.org ↵
- U.S. Energy Information Administration, 2022 ↵
- As you will find throughout this book, here is very succinct summary of the major themes and conclusions of Chapter 10 distilled down to a few sentences and fit to be printed on a t-shirt or posted to social media. ↵
- These questions should not be viewed as an exhaustive review of this chapter; rather, they are intended to provide a starting point for you to contemplate and synthesize some important ideas you have learned so far. ↵
Formally, energy is the capacity to perform work. It enables changes to be made to matter. For example, energy allows you to move a box from the floor to a table. See Chapter 4 for more.
A term related to energy and the capacity to do work. It can be derived mathematically: it is the amount of energy transferred per time unit. Conceptually, power allows a certain amount of work to be done in a certain period of time. It can be expressed with several different units, including the watt. A power generation plant receives thermal power from the burning of a fuel like coal and releases mechanical power in the form of electricity. See Chapter 10 for context.
In simple terms, describes how energy conversions must always go from higher to lower grade, i.e., conversions always lead to degradation of energy. See Chapter 4 for more.
Stored energy. See Chapter 4 for details.
Energy in motion. See Chapter 4 for details.
In ecology, all of the living and non-living entities present in a defined space. See Chapter 5 for more.
An organism that uses the sun as its energy source. See Chapter 5 for more.
A gas made up of one carbon atom and two oxygen atoms, it is one of the many forms carbon can take. This gas is the product of aerobic respiration as well as combustion of fossil fuels, wood, and other materials containing organic carbon. It is not directly usable as a carbon source but can be fixed into useable forms of carbon, for example glucose, by autotrophic organisms. See Chapters 4 and 14 for more.
In chemistry, an adjective that refers to chemical compounds that consist of at least one C atom that is bonded to at least one H atom. Compare to inorganic. See Chapter 4 for details.
In chemistry, refers to chemical compounds that lack at least one of the following: a C atom and at one C-H bond. Compare to organic. See Chapter 4 for details.
An adjective referring to an organism that uses dioxygen in its metabolism. "Aerobe" is the noun, the organism itself. Contrast with anaerobic. See Chapter 1 for more.
One of Earth's four sphere. This term refers to the brittle, outer layer of the Earth made up of the entire crust and the upper portion of the mantle. It is nonliving and lies on top of the asthenosphere. See Chapter 3 for more.
An adjective referring to organisms that do not use dioxygen as part of their metabolism. Many such organisms die in the presence of oxygen gas. "Anaerobe" is the noun. Compare to aerobic. See Chapter 1 for more.
An important fossil fuel, it is mostly comprised of gaseous methane. See methane for more. See also Chapter 10 and 13.
A general word that refers to the breaking down of relatively large, complex materials into relatively small, simple materials. Certain organisms, known as decomposers, break down the remains and waste products of other organisms and use those products as nutrient sources. Decomposers are critical to the health of ecosystems because they aid in the recycling of materials. Fungi and some bacteria are important decomposers. See Chapters 4 and 5 for more.
Refers to organisms that are too small to be seen without the aid of some kind of magnifying lens (e.g., a microscope). Includes bacteria, protozoa, certain forms of algae and fungi, and viruses. See Chapters 1 and 5 for more.
Clean up or repair of an existing problem. See Chapter 15 for more.
On geology, one of the three rocks types. These are formed from weathering products of existing rocks. See Chapter 3 for more.
Barriers to the movement of organisms can effectively cut up a large habitat into small sections. Highways, railways, and pipelines are among the structures that can fragment habitat. In some cases, it can endanger organisms. See Chapter 6 for details.
Reservoirs of oil and natural gas that are locked in the pores of shales and other hard-to-access geologic structures are said to be "tight". They can only be recovered using hydraulic fracturing or other unconventional method. See Chapter 10 for more.
Refers to nations that have relatively small amounts of technology, wealth, and infrastructure compared to the developed world. See Chapter 8 for details.
There are several different types of coal arranged according to their age and energy content, that is, rank. See Chapter 10 for more.
The layer, or soil horizon, that contains the most soil organic matter (SOM). It is is at or close to the surface. This is the most fertile part of the soil profile and is where plants grow; when it is lost, the remaining horizons cannot support plants, including crops. See Chapter 9 for details.
A general term related to biogeochemical cycles. It refers to any process that increases the biological availability of an atom. See Chapter 4 for details.
A pathway of the hydrologic cycle. Water flows across the surface of the Earth; it moves with gravity and follows the contours of the surface. See Chapter 4 for more.
In ecology, the sequential replacement of dominant species in an area; it is mechanism by which an ecological community comes into being. See Chapter 5 for details.
Atoms with the same number of protons but a different number of neutrons in the nuclei. For example, U235 and U239 are different isotopes of uranium. Each has 92 protons (as well as 92 electrons), but the former has 143 neutrons and the latter has 146 neutrons. See Chapter 4 for details and Chapter 10 for some relevance and context.
A term that refers to objects that are smaller than and associated with atoms. These include neutrons, protons, and electrons. See Chapter 4 for more.
Thin tubes packed with fissionable material such as uranium atoms. These rods undergo a chain reaction inside a nuclear reactor. See Chapter 10 for more.
A process carried out by certain organisms (plants, algae, and some bacteria) in which the energy of the sun is used to drive the fixation of carbon. See Chapters 4 and 5 for more details.
An adjective that refers to organisms that live primarily on land (i.e., not in water). Compare to aquatic organisms. See Chapter 4 for more.
In ecology, refers to the specific identity of organisms present in an ecosystem. This property varies widely among ecosystems. See Chapter 5 for details.
One of the processes carried out by organisms in an ecosystem. Important functions include C fixation, decomposition, and consumption. See Chapter 5 for details.
A kind of alcohol that is generally produced through the fermentation of plant materials like corn or sugar. Ethanol is an important ingredient in alcoholic beverages like wine and beer and can also be used as a liquid fuel. See Chapter 10 for more.
A pathway of the hydrologic cycle. Water flows vertically downward due to gravity. See Chapter 4 for more.
A group of organisms that are eukaryotic, phototrophic, and autotrophic. Some are unicellular, but most are colonial. Note: some scientists include certain prokaryotic cells in this group, the so-called "blue-green algae"; others classify those prokaryotic organisms as bacteria (known as "cyanobacteria"). We will follow the latter convention in this book. See Chapter 3 for more.