9 Agriculture, Soil, and Food
Jason Kelsey
Now we turn our attention to the ways we feed the people we just saw in Chapter 8. The concerns of Chapter 9 are intertwined with many other topics in this book as well, including ecology (Chapter 5), water quality and availability (Chapters 4 and 11), energy use (Chapter 10), air quality (Chapter 14), and environmental toxicology (Chapter 15). Put another way, an understanding of agriculture is critical to the study of environmental science. It also should be of interest to anyone who has ever eaten a meal.
Key concepts
After reading Chapter 9, you should understand the following:
- How and why agriculture is employed to produce human food
- How farms are different than natural ecological systems
- The processes that contribute to soil formation and why soil properties matter to farmers
- How maximum sustainable yield is like carrying capacity (Chapter 1)
- The multiple environmental effects of agriculture
- The potential risks and benefits of the genetic manipulation of agricultural organisms (i.e., the use of GMOs)
- The many different approaches to farming used by modern humans to produce food
- How the issues of human population growth (Chapter 8) are intimately linked to those of agriculture and food
Chapter contents
9.1. Agriculture is Integral to Modern Human Societies
9.2. Farmers Harness Ecosystem Functions
9.3. Food Production Relies on Knowledge of Soil Science
9.4. Environmental Effects of Agriculture
9.5. Genetic Modification of Organisms
9.6. Applying Our Knowledge: Approaches to Agriculture
9.7. What is the Future of Agriculture?
The Chapter Essence in Brief
9.1. AGRICULTURE IS INTEGRAL TO MODERN HUMAN SOCIETIES
Agriculture currently dominates food production for humans, but we have not always been farmers. Much evidence suggests that large-scale cultivation of crops began about 12,000 years ago, meaning earlier peoples fed themselves through hunting and gathering. The development of agriculture had a profound effect on human civilization because, among other things, it allowed people to settle in one area rather than moving from place to place, in a nomadic fashion, searching for food. In addition, it broadly increased food availability. The large-scale farming of the past hundred years or so also has brought on adverse consequences. Do the benefits of agriculture justify its costs? What would we do without it? Might we come up with a better system? You can wrestle with those and other important questions as you proceed through this chapter.
9.2. FARMERS HARNESS ECOSYSTEMS FUNCTIONS
An incomplete, but useful, definition of agriculture is: the manipulation and augmentation of natural ecological processes by humans to concentrate and maximize the growth of food in areas that would otherwise be less productive. Employing terminology common in agriculture, farmers use available tools and resources to optimize crop yields, the amount of food produced per acre of land. Additionally, large numbers of animals are concentrated and raised so they (or their products) can be consumed by humans. Unlike the hunter-gatherers mentioned above, who had to search—sometimes by traveling great distances—to secure enough to eat, in a sense we have learned to make our food come to us.
9.2.1. How food is produced on farms: a brief overview
Although specific details can differ substantially from farm to farm, an essential set of steps is usually followed when crops and animals are raised by humans. A brief overview of these processes is provided here, but we will explore more details about agriculture and food production later.
Crops
Existing ecological communities such as forests are cleared to make room for crops. The upper portion of soil is typically loosened by plowing or a similar process, and then seeds are planted. If deficiencies are noted, nutrients and water are applied to agricultural fields. Additionally, chemical pesticides may be added or other steps taken to inhibit unwanted organisms that may interfere with productivity. When they are mature, crops are harvested.
Livestock animals
Animals are bred on a farm or at another facility specifically designed for that purpose. Food grown on the farm or elsewhere is given to animals to fatten them up, and certain growth hormones and antibiotics may be added to their diets. Animal waste products must be managed to prevent soil and water pollution, sometimes by using them as fertilizer for crop growth. In the cases where products such as milk and eggs are sought, they are collected and processed. Ultimately, nearly all animals are slaughtered when they reach a target age.
9.2.2. Farms are agroecosystems
Environmental scientists often use the term agroecosystem because, although they do take advantage of natural processes, farms differ from naturally occurring, undisturbed, ecosystems in several important ways.
Stunted ecological succession
Crops like corn, wheat, and hay resemble early stage successional species more than they do late ones (Chapter 5), so farmers tend to clear land and maintain it in that open condition indefinitely. Since agriculture is most common (and successful) in temperate terrestrial areas, allowing succession to proceed unimpeded would ultimately lead to a climax community dominated by tall, shade-tolerant trees, an environment that discourages crop growth. Unfortunately, artificially preventing succession in this biome also provides an inviting environment for unwanted plants—known, of course, as weeds—because they too thrive in sunny, barren areas (weeds are typically fast growing and grassy). In short, by creating conditions favorable to the plants we want, we encourage the growth of costly competitors as well.
Monoculture
To maximize efficiency, most farmers employ the practice of monoculture: just one crop is sown on any parcel of land. A farm is likely to use several different fields at one time to grow, say, corn, hay, and soybeans, but the species are kept separate from each other. On the other hand, as we saw in Chapter 5, natural ecosystems consist of a community of different organisms (Figure 9.1 compares a corn field to a natural forest).
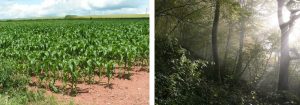
In addition, in an agroecosystem, plants tend to be packed together far more densely than they would be under natural conditions. Two unintended and unwelcome consequences result from the practice of monoculture. First, insects adapted to eat the cultivated crop are provided with a substantial advantage. Since their food source is highly concentrated in a field, nutritional limitations are no longer a problem. They can get on with the energy-intensive and fundamental business of reproduction (Chapter 5). For example, grasshoppers can live on several types of plants, including corn. Imagine yourself to be such an insect and what it must be like to happen upon a 500-acre corn field after struggling your whole life to find food in diverse ecosystems characterized by scarce resources. One of your major worries would be over! Second, a high concentration of one plant species in an area quickly depletes the soil in which it grows of necessary nutrients.
Plowing
This and related practices facilitate seed germination by physically breaking up hard, compacted soil prior to planting. Plowing also enhances water movement through soil and can damage the roots of weeds. Despite the benefits, though, mechanical disruption of soil does have quite a downside: among the adverse consequences of plowing is the way it greatly accelerates the loss of fertile soil from farmlands. Topsoil erosion is a major concern associated with agriculture, and we will devote substantial time and energy to it later in this chapter.
Manipulation of nutrients and water
Recalling that the purpose of agriculture is to produce as much food as possible in a given space, it ought to stand to reason that farmers do everything they can to stimulate growth of crops. For example, nutrients exhausted through excess demand by crops and lost when topsoil is eroded can be artificially replenished through the application of fertilizers, mixtures containing biologically available forms of nitrogen, phosphorus, and other elements (Chapter 4), to fields. Natural ecosystems have no such means to quickly replace what is missing. Fertilizer use can provide a lot of benefits, but we will see how it can also lead to pollution of water (later in this chapter and Chapter 11). Water supply on farms can also be supplemented through the addition of water to fields in ways that are not applicable to natural systems. This practice, known as irrigation, can be the difference between success and failure of crops, but it requires the costly transport of water to farms from other regions. The environmental, political, and economic consequences of irrigation can be substantial, as described later in this chapter and in Chapter 11.
Inhibition of unwanted organisms
It is important to realize that farmers do not want organisms other than crops (plus any organisms that might enhance crop growth) to succeed in their fields. They can use one or more chemical, biological, and physical strategies to discourage pests that interfere with crop production (more in an upcoming section of this chapter). There are some analogous processes in natural ecosystems, as certain organisms in natural ecosystems possess chemical and other weapons to fight competitors (Chapter 5), but the scale on which they are deployed is much larger on farms.
Minimal recycling of nutrients
On many farms, crops are completely removed at the end of a growing season. In other words, in addition to the target product (ears of corn, the soybeans themselves, etc.), the entire plant is cut down and taken away rather than being left in the field. This practice clears a field for future planting, but it does not take advantage of natural decomposition and the way it helps return nutrients to the soil for next-year’s crops. Natural ecosystems are very different because cycling and recycling of nutrients is integral to their functioning and continuation (Chapters 4 and 5). It is worth noting here and will be reiterated below that some farmers do in fact leave plant residues behind in fields after harvest, but that practice is not widespread.
9.3. FOOD PRODUCTION RELIES ON KNOWLEDGE OF SOIL SCIENCE
It tends to be taken for granted by most people, but soil is a vital resource that merits special care, preservation, and study. We are interested in it as environmental scientists for at least four reasons. First, with very few exceptions, crops are grown in soil. Our ability to effectively farm is directly related to soil quality: agriculture is successful in soil that is characterized by a certain set of necessary features and properties—put simply, if it is fertile—and fails in the wrong kind of soil. Importantly, though, the very practice of agriculture tends to reduce soil quality, that is, the more it is used for farming, the less able to support crop growth it becomes. Second, the ecology of soil affects all of Earth’s systems. Even the tiniest soil organisms are indispensable to the cycling and ultimate availability of nutrients to the rest of the biosphere. Third, pollutants released into natural environments are often modified by processes occurring in soils (we will return to this topic in Chapter 15). Finally, the building of houses, bridges, and other structures is influenced by the chemical and physical properties of soils. Put into stark terms, whether a new bridge stands or falls after it is exposed to its first intense rainstorm is in part a function of the way the soil beneath it behaves. Since knowledge of soil science informs many activities and processes valued by humans, including food production, we will take some time here to study its basic principles.
9.3.1. The definition of soil
Environmental scientists define soil as a naturally occurring material that meets the following criteria.
It is unconsolidated
Unlike rocks, the component parts of soil are not cemented together into a solid object. They are loosely packed and can be easily moved, changed, or transported by wind, water, and ice.
It comes from other materials
We will study its formation in more detail shortly, but for now recognize that soil is produced through biological, chemical, and physical modifications of pre-existing organisms and rocks.
It contains solids and spaces
Soil is referred to as a porous medium because it is a matrix of solid materials and pores.
It is layered
As we will see below, to be classed as soil, unconsolidated material must be arranged in characteristic layers which differ from each other in important ways.
It can provide nutrients, habitat, and water for organisms
Soil supports the growth of a diverse array of plants, animals, and microorganisms.
9.3.2. How soil is formed
Soil formation begins when existing organisms and rocks, known as parent materials, are first exposed to forces such as weathering and decomposition. The resulting products undergo additional modifications, and recognizable soil is eventually created. Note that the development of soil often requires decades or centuries and that even after it comes into being, soil continues to be modified by the same forces that initiated its production. The chemical and physical properties of any soil will therefore change as the soil ages. Figure 9.2 provides a simple diagram of soil formation.
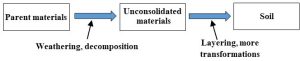
Weathering and decomposition of parent materials
Rocks. Rocks are consolidated objects largely comprised of inorganic chemical compounds. They can be broken down into small fragments by both physical disintegration and through a number of chemical reactions (Chapter 3). These products, known as unconsolidated sediments, are not soil by our definition because they lack the layering referred to in Figure 9.2, above. Given the appropriate conditions, additional modifications yield true soil.
Remains and products of organisms. We have seen elsewhere (Chapters 4 and 5) that decomposers are integral to nutrient cycling because they break down the dead tissues and waste products left by organisms. They are also critical in soil formation because they process and convert these remains into an important material known as soil organic matter (SOM). The chemical and physical properties of SOM are extremely complex and not even completely understood by soil scientists (this is an area of current research). We define SOM by several characteristics.
It originated from organisms
SOM is primarily made up of chemical compounds produced by living things.
It contains materials from multiple organisms
The remains and products of microorganisms, plants, and animals are mixed together and collectively become SOM. Many forms of carbon, nitrogen, phosphorus, and other nutrients are present.
It does not resemble organisms in appearance
Although SOM does come from living members of the biosphere, it does not contain recognizable structures such as leaves or body parts. It is instead comprised of chemical compounds that have been dramatically altered. Some of their inherent identity is intact, but many old chemical bonds are broken and new ones formed as the materials are processed by decomposers. It could be visualized a bit like soup you might start from vegetables, meat, and spices on Monday but then forget about and leave cooking until Sunday. When you get back to it days later, it is likely to be a uniform mush: the distinct ingredients will have been blended together, making it difficult or impossible to recognize them in their original forms. The whole thing is now a new entity with large-scale properties, not a collection of individual pieces of carrots, chicken, and the like floating in water. So, the remains and excrement of fungi, grass, rabbits, bacteria, foxes, and all the rest that live in an area get transformed and combined to form SOM (Figure 9.3).
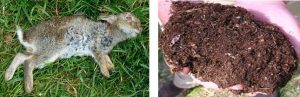
Development of layers
The relatively uniform accumulation of weathering products from the first step in soil formation can be slowly arranged into layers due to several processes, two of which are briefly described here (see Figure 9.4, an idealized overview of the process).
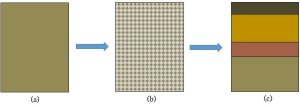
Removal and re-deposition. Some materials in the upper portion of unconsolidated sediments are easily removed by flowing water and carried vertically downward (a process known as leaching—see Chapter 11). Many of these materials leave the water after they have travelled to some depth and end up redeposited into the matrix of sediments. As a result, the properties of both the upper and lower layers are changed.
Incorporation of SOM. Remains and products of organisms accumulating on top of the soil begin to undergo decomposition and transformation to SOM as described above. As time goes by, more and more of this organic material is incorporated into the soil layers near the Earth’s surface, combining with the inorganic weathering products that originated in rocks. Microscopic examination of soil reveals patches of SOM adhering to larger surfaces of the inorganic material in soil (Figure. 9.5).
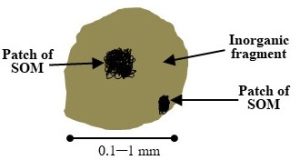
The soil profile and soil horizons
The soil profile is a cross-sectional view of the true soil produced by the differential gains and losses of materials in upper and lower regions, the additional of SOM, and some other chemical changes. It contains several layers, known as soil horizons, that are each characterized by distinct chemical, physical, and biological properties. Generally, top horizons contain SOM and are the zone in which plants, including crops, and other organisms can grow. Bacteria are the only members of the biosphere found in the lower layers. Specific properties, including thickness of individual horizons, can vary widely from location to location. Sometimes, certain horizons are completely absent. The major horizons and their properties are summarized in Table 9.1, and Figure 9.6 is a photo of an actual soil profile (note the photo is not as ideal as is suggested by Table 9.1).
Horizon | Important properties | Typical depth (cm) |
---|---|---|
O | Contains both identifiable and partially decomposed remains of organisms | Surface to a few cm |
A | Consists of topsoil; high concentration of SOM; brown / black in color | 5 — 30 |
E | Inorganic materials; lacks SOM; light color | 10 — 60 |
B | Known as subsoil; various colors, can be darker than E; accumulation of materials leached from A and E | 30 — 80 |
C | Partially weathered parent rock | 60 — 100 |
R | Solid bedrock | Below C |
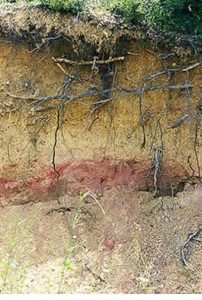
9.3.3. The composition of soil
Soil is made up of solids and pores, each of which plays important roles for crops and other organisms.
Solids are derived from parents
Mineral fraction. Also known as the inorganic fraction, this is the bulk of a soil. It is produced by weathering of rocks, and its composition varies with the chemistry of those parents. It provides the solid support matrix of soils and some inorganic nutrients.
Organic fraction. Soil organic matter generally accounts for 5% or less of the weight of a typical agricultural soil, although it can range from 0 to 90% among soils. Its chemical properties depend on the nature of its parent materials and can vary widely. In soil it provides several important services.
Nutrition
As we know, SOM was produced from organisms so contains a lot of biologically available nutrients.
Structure
SOM provides support to a soil by acting as a kind of glue—it helps hold the mineral fragments together and allows for the formation of large clumps, referred to as aggregates, of soil (sizes of 10 or more cm in diameter are possible—See Figure 9.7).
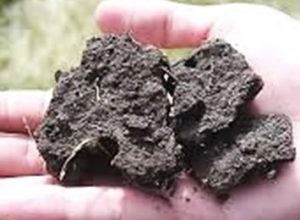
Water retention and entry
The structure provided by SOM helps create openings through which water can move into and be held by soil. As we will see, the amount of water present in soil is a critical property affecting crop growth.
Retention of toxins
The chemical properties of SOM make it an ideal place for certain chemical pollutants to bind. See Chapter 15 for more.
Pores are spaces among the solids
Open spaces within the soil matrix can hold water and air. These two vital substances vary in relative abundance as a function of specific properties of a soil as well as climate and weather.
Water. Some fraction of the pore space within a soil will be occupied by water. The amount found here is influenced by the amount of SOM present as well as the sizes of the particles in a soil (described in detail in the section below).
Gases such as air. In most surface soils, atmospheric air is the gas stored in pores. It can provide much-needed oxygen, carbon dioxide, and dinitrogen for various organisms. The amount of water and air in soil are related to each other: as one increases, the other necessarily decreases.
9.3.4. Important soil properties
The chemical and physical properties of any soil are a function of a number of variables, including the chemistry of the parent materials, the climate in which the soil was formed, biological activity, and the age of the soil (more below). In other words, given that each came into being under different conditions, thousands of different soils exist. Several characteristics are used to identify and distinguish soils from one another. These properties are also important in determining the suitability of a particular soil for crop growth. Here we explore a small number of those most relevant for agriculture.
Texture
The solid portion of soil is comprised of particles that are grouped according to their size: clay (diameter below 0.002 mm), silt (0.002 – 0.05 mm) and sand (0.05 – 2.0 mm). The relative proportion of these size fractions, known as soil texture, can be determined through laboratory tests and is used to assign soils to different categories. According to the United States Department of Agriculture (USDA), there are twelve major soil classes: those at the three end points (ideally, 100% sand, soil, or clay) and nine intermediates[1]. For example, “loam”, consisting of approximately 40% each of sand and silt and 20% clay, refers to a soil commonly used in agriculture. Texture is an important property because it influences the way water flows through soil. Too many very small particles (i.e., clay) will inhibit movement of water, likely flooding plants, whereas too many large particles (i.e., sand) will allow water to drain from soil too quickly for plants to access it.
Soil organic matter content
The percentage of the weight of a soil that is made up of SOM can be readily determined in a laboratory. As noted above, a very wide range of SOM contents is possible. Like most things we have encountered, both too much and too little of this vital constituent can inhibit plant growth and farm productivity. An overabundance of it will hold too much water in soil, and a deficit will deprive organisms of the services provided by SOM that are listed above.
Water-holding capacity
Soils differ in their ability to store water, and this important property is a way to quantify those distinctions. Three points related to this concept merit our brief attention.
Water remains after inputs cease. During a rainfall event water is freely available and moves vertically downward through the soil. Once the precipitation stops, water continues to drain for a brief period, leaving behind some amount of water stored inside pores. The amount of water remaining is essentially a soil’s water holding capacity.
Water holding capacity affects plants. Water held in pores is very important because it can be used by plants to meet their needs for an extended period, potentially many days or weeks into the future. Under the right circumstances, plants will get what they need from this stored water until it rains again. Plants will eventually wilt and die, however, if dry conditions persist.
Water-holding capacity varies. The capacity to store water depends on soil texture and SOM content, two properties that differ among soils. As we saw, abundant clay or sand will retain either too much or too little water, respectively, for healthy crop growth. Organic matter in soil is also critical: as its content increases, so does water-holding capacity. In short, some soils are simply unsuitable for agriculture because they do not provide the proper amount of water to plants.
Chemistry
Several measurable chemical parameters influence crop growth. We will only consider two of the most important ones, but you should recognize that many others play roles in agriculture.
Nutrients. As we have seen previously, the amount and type of useable nutrients such as nitrogen and phosphorus present are extremely important. Farmers can use various tools to assess nutrient content and make up for deficits they might discover (for example, by using fertilizers).
Acidity. Another critical concern is the pH, or acidity, of a soil. Very briefly, the concentration of hydrogen ions (routinely expressed as H+) present in water can be measured, and that number converted to a unit known as pH. The pH scale is generally understood to run from 0 – 14, with the lower numbers representing the most acidic substances and the higher numbers representing the most basic (or least acidic). A pH of 7 is neutral, neither acidic nor basic. Soils vary in their pH values, so their ability to support crop growth varies as well. Not all plants thrive at the same pH, and growth can be seen at acidic, neutral, and basic conditions. Farmers can determine acidity and then adjust it to some extent if necessary. We will encounter pH again in Chapter 14 when we study air pollution, including the way acidified precipitation can affect soil quality.
Color
This property is a function of soil composition. For example, dark brown or black soils contain more SOM than do lighter soils, red soils are rich in a certain type of iron, grey and yellow soils have different types of iron in them (Figures 9.8.a. and 9.8.b). Color alone cannot provide enough information to completely characterize a soil, but it is used to estimate the likelihood that a soil can be farmed effectively.
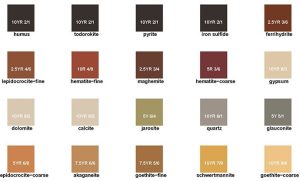
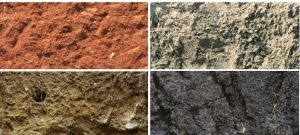
Limiting factor
Of all the requirements for growth, sun, space in soil, water, carbon, nitrogen, phosphorus, and other chemical nutrients, the limiting factor is the one item least available relative to demand for it. Imagine a field full of stunted corn, plants that barely produced a few ears. The answer to the question “why did this crop fail to grow as anticipated?” is, by definition, the limiting factor. So, a farmer might investigate what happened and discover everything needed for optimum corn growth is available in sufficient amounts except for nitrogen. That nutrient may be added to the soil for the next season (or in the current season, if the problem is detected early enough) and yield should improve. The concept of limiting factor can be applied to a wide range of ecological systems, not just farms, to determine why organisms and communities are not as successful as might be expected. For example, despite abundant sunlight, CO2, appropriate temperature, and other favorable conditions, the open ocean (i.e., far from a coastline) supports a relatively small number of organisms. What limits growth in this otherwise hospitable environment? Often, it is lack of phosphorus, nitrogen, or iron.
Soil fertility
Soil fertility is defined as the ability of a soil to provide necessary nutrients to support crop growth into the foreseeable future. Realistically, though, since we know that repeated farming tends to reduce soil quality, productivity is likely to deteriorate with time as well. Among the important reasons for this phenomenon is that nutrient availability declines as soils age. In fact, a crucial difference between young and old soils is diminished fertility in the latter relative to that of the former.
Maximum sustainable yield
This final property is really a function of the others on this list and is a critical consideration for farmers. Maximum sustainable yield is the largest amount of a crop that can be produced in a given season without reducing the ability of that field to produce the same amount of that crop in subsequent seasons. Although it might be possible to produce an enormous quantity of food for one or two years, the soil will lose so much of its fertility as a result that crops will be less successful in the future. The goal is to strike a balance that allows for long-term yields to be as even and predictable as possible. This property should seem familiar to you as it is closely related to the concept of carrying capacity we encountered in Chapter 1. In this case, though, it is applied specifically to agroecosystems.
9.3.5. Soil properties depend on environmental conditions
It likely makes sense to you that the physical and chemical characteristics of parent materials affect the soil that results from their modification: the nature of the outputs from any process will be closely tied to the nature of the inputs that are available to build them. However, like all reactions, the conditions under which parents are weathered and otherwise transformed also play a critical role in shaping the resulting soil. For example, soils produced in hot, wet environments like the tropics are different than those from cool, dry, polar regions. How much oxygen is present will also influence the forms of iron and other elements in soil. Furthermore, the specific identity of the organisms involved in decomposition is not constant from place to place, so the products of their activities will be similarly different. In short, the properties of parent materials alone cannot be used to predict the nature of the soils produced from them.
9.4. ENVIRONMENTAL EFFECTS OF AGRICULTURE
Employing agriculture to produce food on a scale large enough to meet current demand comes with consequences. As we will see, these adverse effects are relevant at three scales: local, those that occur on the site of a given farm, regional, those that influence surrounding areas that are not farmed, and global, those that affect the entire planet.
9.4.1. Increased rates of soil erosion
Arguably, the rapid loss of fertile soil is the most important effect of agriculture because of the relationship between soil quality and food production noted earlier. It is generally observed on a local scale because it affects fields that are actively farmed; it is very much a problem around the world, though. Put another way, activities in, say, North America do not directly bring about soil erosion in a place like Africa, but loss of soil is still of great concern on both continents.
Causes: agricultural practices
Land clearing. Earlier in this chapter we saw how existing ecological communities are typically removed prior to farming. Unfortunately, loss of vegetation leaves an area vulnerable to erosion because plants help to hold soil in place in two important ways. First, living roots create a network that physically stabilizes soil, as in sand dune succession (Chapter 5). Second, above-ground structures like leaves and branches can block or deflect incoming rainfall, preventing it from hitting the ground directly. Thus, soil is protected from the full force of the weathering power of water.
Plowing. As we saw earlier, this common practice can aid germination and growth of certain crops. However, plowing breaks large and relatively heavy aggregates into small and light particles, greatly increasing the likelihood that wind and water can easily carry them away (Figure 9.9).
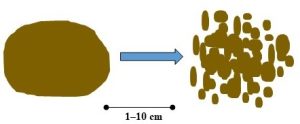
Overgrazing. Farm animals often supplement their diet by eating grasses growing naturally in fields. The trouble starts when their grazing removes the plants faster than they can re-grow, or put another way, when carrying capacity of the field is exceeded. The outcome is essentially the same as that seen after the deliberate clearing of land: cows (or others) rip the grass out of the ground, leaving behind loose soil that can be easily removed during the next rainstorm (Figure 9.10).
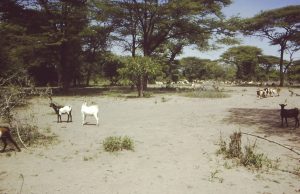
Furthermore, overgrazing changes the structure of the ecosystem in a field because it favors plants that can quickly grow in response to cleared land. Reduced biodiversity is typically a result (Chapter 6), because few organisms possess the traits necessary to thrive under such conditions.
Relevance: loss of topsoil and reduced soil fertility
Topsoil erosion is a natural part of the rock cycle (Chapter 3), and materials weathered, transported, and redeposited can be incorporated into new soils as described in section 9.3. Under natural conditions, these processes balance each other sufficiently to ensure no net change in topsoil availability. Farming, however, has dramatically accelerated the pace of losses, and global rates of erosion are currently many times those of replacement[2]. Drawing upon the symbology and concepts from systems analysis (Chapter 2), we can conclude that agricultural systems are experiencing a net loss of topsoil (Figure 9.11).
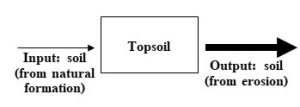
This trend is problematic for farmers and limits food production because topsoil is the only layer in the soil profile that contains relevant amounts of soil organic matter. Thus, as topsoil is lost, SOM and the services it provides (described in section 9.3) are lost as well. Note that ( positive feedback only makes matters worse (see Chapter 2) because of the ways SOM helps soil resist erosion. In other words, as it is removed, the rates of its future removal will increase. Eventually, soil fertility drops so low that crops will no longer grow.
How to minimize loss and conserve soil
The United States Department of Agriculture, along with analogous state agencies, has developed a set of formal guidelines farmers can follow to help preserve their soil. Many other countries promote similar practices. The strategies described below are a sampling of recommended soil conservation methods that could, if widely adopted, dramatically reduce rates of erosion.
Contour farming. Cultivation on the side of a hill can slow the downward movement of water and sediments if crop rows are perpendicular to the direction of the slope rather than parallel to it (Figure 9.12).
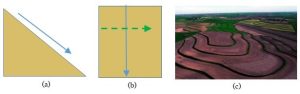
Cover crops. After a crop such as corn or wheat is harvested, a field may lie empty for many months, leaving its soil vulnerable to erosion. To combat this problem, another plant can be sown in the field after the main crop has been harvested. There are at least two important advantages of this practice. One, the second plant helps hold the soil in place in the offseason. Two, some nutrients necessary for future crop production can be replenished if certain cover crops are used. For example, clover or alfalfa are often chosen because they house nitrogen-fixing bacteria inside their tissues (Chapter 4). This mutualistic association (Chapter 5) is extremely important because the host plant receives abundant useable nitrogen, and the bacteria are given a suitable habitat and fixed carbon (Chapter 4). Since the mature plants are generally plowed into the soil rather than harvested, decomposition will make that fixed nitrogen available for the food crop planted in the next growing season.
Windbreak (or shelterbelt) establishment. Rows of trees can be strategically planted near crop fields to help block wind and reduce the soil erosion it causes (Figure 9.13). The presence of trees can also add to the biological diversity of an area, which could help combat insect pests (more below).
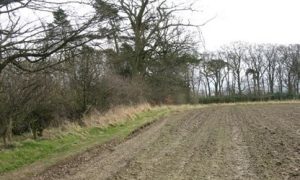
No-till farming. So far in this chapter we have assumed agroecosystems utilize mechanical plowing or tilling as a matter of course on all crops. However, an increasing number of farmers (but still less than a majority) choose to sow seeds in fields that have not been disturbed or turned over. Their crops are grown on top of plant residues from the previous year (Figure 9.14 shows a field plowed prior to the sowing of seeds and a field that was not plowed).
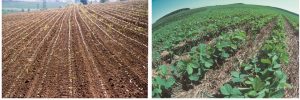
The advantages of this practice are likely obvious by now: soil will be less vulnerable to erosion if its aggregates are not broken down into small fragments. There also are disadvantages. As we saw above, plowing can enhance crop growth. Furthermore, pest management becomes a particularly important concern in no-till agriculture, often necessitating more pesticide use (we will return to this topic soon). Since fertile soil is a limited resource, though, one that is being diminished faster than it is replaced, more and more farmers may elect this approach.
Our second adverse consequence is directly linked to the first, soil erosion. What happens to all the soil carried away from farms by wind and water? Surely it does not just vanish, so where does it go? In short, much of that soil ends up moving into bodies of water (and called “sediments”), bringing about substantial changes to those aquatic ecosystems. This effect of agriculture, referred to as sediment damage, can be observed on both local and regional scales. Eroded soils pollute the small streams that often flow across farmland and then move hundreds or even thousands of kilometers to rivers, lakes, and oceans.
Causes
The practices that drive the removal of soil from agricultural fields are also responsible for sediment damage. So, land clearing, plowing, and overgrazing all cause an excess of sediments in waterways.
Relevance
Sediment damage reduces water quality. As we have seen repeatedly, organisms in any environment are there because they have adapted to the pressures placed on them. Fish living in a river, for instance, are accustomed to the clarity and temperature of their water as well as the amounts of oxygen and other vital materials in their habitat. Alterations to any of those characteristics will favor different organisms. Two specific concerns are briefly described here.
Increased turbidity. Water becomes more turbid, that is, cloudier, when sediments are added to it (Figure 9.15). Many changes can then take place. First, less sunlight penetrates the water, which reduces rates of photosynthesis (Chapter 4). Among other things, less oxygen will be released into water, challenging or killing aerobic organisms. Second, fish sensitive to the stress of more physical objects passing over their gills will begin to die. Third, the added sediments absorb heat, leading to higher water temperature. This last change drives even more oxygen out of the water and it puts pressure on organisms that have become adapted to lower temperatures. Under extreme conditions, water quality becomes so poor that virtually all fish disappear from an aquatic system, leaving behind certain insects, worms, microorganisms, and other organisms adapted to life in murky, polluted water.

Increased amounts of nutrients. Soil contains many nutrients, including biologically available forms of nitrogen and phosphorus. Eutrophication may therefore result from sediment damage.
How to minimize sediment damage
Recalling that this effect is brought about by soil erosion, it is fair to say that the soil conservation methods described above would also reduce sediment damage. Prevention, in other words, is an effective way to preempt the problem.
9.4.3. Chemical pollution
Conventional agriculture generally employs chemical substances, collectively referred to as agrochemicals, to maximize crop yields with a minimal financial cost. Two basic types of chemicals are used on farms: stimulants and limiters. As we will see, each type of additive can cause local and regional effects. Before we proceed, though, the following clarification might be helpful. The sediment pollution described in the previous section is also termed physical pollution because our primary concern with it is linked to the presence of objects floating in the water. Alternatively, chemical pollution describes compounds that are largely dissolved in water (or other relevant medium) and typically invisible to the naked eye.
Fertilizers stimulate plant growth
Recall that organisms require energy and materials to survive, grow, thrive, and reproduce. Like all plants, crops are photosynthetic, so neither fixed carbon nor energy are likely to be limiting. However, other critical compounds, for example those containing nitrogen, phosphorus, and potassium, are often available in only very low quantities. These deficiencies can be addressed by addition of fertilizers, substances rich in nutrients. Here we briefly consider some of the ways this practice is used to stimulate the growth of desired crops.
Types. Conventional farms often use synthetic fertilizers, that is, those that have been manufactured, processed, and packaged in laboratories and factories. In other cases, compost or animal manure can be applied to fields. This second strategy is possible because, as we already learned, waste products released from organisms contain a lot of useable nutrients.
Benefits. Fertilizer can have an enormous impact on crop yield. Without it, growth can be stunted and food production low, but, if added appropriately, growth can be successful and profitable.
Costs. Fertilizers are generally helpful, but their use can also lead to many undesirable outcomes.
Water pollution
As will be described in more detail in Chapter 11, agriculture can contaminate both human drinking water and natural waterways. Although it might seem odd that necessary nutrients like nitrogen can be harmful if they end up in water, you should be aware that the types of nitrogen added to fields are toxic to animals. Moreover, once they enter soil, some of these compounds can be converted into forms that are even more problematic, which then move vertically to the groundwater reservoir with flowing water (Chapter 4). For example, blue baby syndrome can arise from ingestion of a natural product of the nitrogen cycle. This condition can interfere with the body’s ability to transport O2 to the brain, causing death in some cases. Eutrophication is another potential consequence of fertilizer use (Chapter 4).
Stimulation of weeds
As we know, weeds thrive in the same kinds of conditions favoring crops. The addition of fertilizers exacerbates the situation because these unwanted plants also use the nutrients to grow more effectively.
They must be purchased
Any list of the costs of fertilizers would be incomplete without a note about a more familiar type of cost, the financial kind. Fertilizers are not free of charge, so a farmer must determine if increases in crop production justify the investment required to provide supplemental nutrients.
Reducing or eliminating fertilizer use. You should keep in mind that the right amount of nutrients needs to be present to support crop growth. Think back to our exploration of biogeochemical cycles in Chapter 4 and recall that there are critical limits of tolerance for chemical elements in any system. The same principle applies to agriculture: insufficient amounts of things like available nitrogen will limit food production but too much will lead to water pollution and cost more money than needs to be spent. With that guidance in mind, we can consider ways to minimize the amount of fertilizer that must be applied to fields.
Nitrogen-fixing cover crops
In the previous section about erosion we saw that certain cover crops can help stabilize a field and minimize soil loss. They can also replenish the supply of available nitrogen compounds for next season’s crops.
Crop rotation
Earlier we noted how monoculture can both stimulate pests and lead to nutrient depletion. One effective way to reduce the severity of those unwanted consequences is to plant different crops in different fields each season. Monoculture is still employed, but this year’s corn field will be used to grow wheat next year, the wheat field will be used to grow corn, and so forth. Ideally, one of the many fields a farmer owns is left fallow, that is, no crop is grown in it at all (except a cover crop that is not harvested) for one season. Crop rotation can reduce the need for fertilizer because, although they all have the same fundamental needs, different species of plants use somewhat different specific amounts of nitrogen, phosphorus and other nutrients. So, if a crop such as corn was grown over and over in the same field for years the nutrients it needs the most would be used up faster than if it and other crops were moved around from year to year (see Figure 9.16 for a hypothetical map of crop rotation for three years).
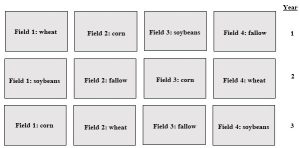
No-till agriculture
The elimination of plowing and other processes that break up soil is another strategy we encountered during our discussion of erosion, above. In addition to reducing soil loss for the reasons already described, this method can minimize the need for fertilizers because remnants of the previous year’s plants stay in the field instead of being completely removed in preparation for upcoming growing seasons. The remains undergo decomposition, and the nutrients stored in their dead tissues are released and made available for the next generation of crops.
Hormones stimulate animal growth
Earlier in this chapter we saw that these chemicals are sometimes given to livestock to promote growth.
Benefits. This practice is used because it can lead to an increase in food production without an increase in the number of animals present. For example, the amount of milk produced per cow goes up if certain hormones are mixed into feed.
Risks. Several adverse consequences are possible, leading some countries, particularly those in Europe, to greatly restrict the use of hormones in livestock; the same regulations do not limit U.S. farmers, though.
Harm to the animals
Data suggest that cows receiving hormones can suffer adverse effects, including abnormal development.
Harm to human consumers
Since hormones play an important role in processes related to growth, development, and sexual function, many people have expressed concern that human diseases could arise from consumption of food containing residues of these chemicals. More research needs to be conducted, but some scientific evidence has linked certain cancers and other disorders, particularly in children, to hormone ingestion (we will see more about human toxicity in Chapter 15).
Harm to natural ecosystems
Waste products from livestock can move into soil and water, carrying hormone residues with them. Both individuals and communities exposed to such chemicals could experience adverse consequences. For example, the presence of hormones in waterways has been shown to disrupt the reproduction of some amphibians (also addressed in Chapter 15).
Reducing hormone use. The financial benefits of hormones make them desirable for some farmers. The alternative is to cease the use of hormones and add more individuals to herds to make up for the difference in production (which would require additional resources, of course). As stated in the previous paragraphs, more scientific research could help guide the development of regulations and practices.
Pesticides inhibit insects and weeds
Unwanted organisms, pests, can greatly reduce the production of food on farms. Estimates vary, but on a global basis, the combination of weeds and insects has the potential to reduce yields by somewhere between 20 and 50% (or perhaps more). Consequently, much effort is devoted to controlling these destructive organisms, including the widespread use of pesticides.
What are pesticides? The Greek suffix “cide” refers to killing, and the prefix “pest” refers to something that is bothersome or harmful. The literal translation of this word, then, essentially sums up what these chemicals do: they cause the death of unwanted organisms.
How do pesticides work? Pesticides rely on the basic principle of selective toxicity described in Box 5.6 (also Chapter 15): they are poisonous to some, but not all organisms. A well-designed pesticide will not affect humans or other organisms we wish to preserve (referred to as non-target organisms) and damage those that are undesirable (called target organisms). We will see shortly that there is a limit to just how selectively toxic chemical pesticides can be, though.
Types of pesticides. Pesticides are classified using several criteria.
Specific type of pests they target
For example, insecticides kill insects, herbicides kill weeds, rodenticides kill rodents, and so forth.
Number of different organisms a pesticide is intended to affect
Pesticides with a broad spectrum are general in their targets, intentionally killing many organisms, whereas those designed to damage a relatively small number of species have a narrow spectrum.
Source or origin
Compounds can be placed into one of two general categories. The first, called synthetic pesticides, includes those manufactured by humans. Atrazine, Roundup, Malathion, Sevin, and DDT are but a small sampling from this group. On the other hand, natural pesticides (or biopesticides) are obtained from organisms, that is, they are not synthesized by humans. For example, pyrethrum is an insecticide produced by plants. Poisons made by fungi and bacteria can be used to kill insects as well. By and large, synthetic pesticides are more toxic to humans and have a broader spectrum than do biopesticides.
Other characteristics
The pesticide story is quite complicated and includes considerations about how to apply them, and when during the season they are most effective, issues that are unnecessary for our discussion.
Benefits. Herbicides kill plants that would otherwise compete with crops, and insecticides kill caterpillars and other herbivores that eat crops. Unrelated to farming, yet important: they also kill insects that carry human diseases (more in Chapter 15).
Risks. Scientists estimate that over 95% of pesticides applied to crops go someplace other than their intended target. Unsurprisingly, then, pesticide use can bring about many undesirable consequences.
Arguably, ours is the non-target species that gets the most attention when it comes to adverse effects of pesticides. Although we are different than plants and insects and should be protected by the principle of selective toxicity, certain herbicides and insecticides still have the potential to cause human disease. For example, because it disrupts plant hormones, you might expect that the widely used weed killer 2,4-dichlorophenoxyacetic acid (known to most of the world as 2,4-D) would be safe for humans. Some scientific studies suggest otherwise, though: 2,4-D may damage human reproduction by disrupting our own hormones. Other herbicides also seem to pose similar risks. The insecticide DDT (more below and in Chapter 15) is another example of a pesticide that ought to be safe for humans because it specifically targets the nervous systems of insects. It is true that we are not affected the same way target organisms are, but DDT can cause a different set of problems instead. Notably, it has the potential to disrupt reproduction and other processes controlled by hormones in humans. Some people suspect it can also cause cancer, but at this time insufficient convincing evidence supports that hypothesis.
One final note is appropriate here. Humans can contact pesticides through multiple means, including the consumption of contaminated food, but workplace exposure is likely the most important. In other words, the amount of pesticide one will encounter on a piece of fruit from a grocery store is usually very small—so low, in fact, that it will probably have no relevant impact (more about the effects of toxins on human health is presented in Chapter 15). However, a person working in an agricultural field can be exposed to dangerously high levels of pesticides. Consumption of contaminated water is another way these poisons can enter humans, a topic discussed shortly.
Other non-target organisms are affected
Along with killing harmful insects that eat ears of corn or foliage of soybeans, an insecticide can also harm beneficial organisms. Many examples could be cited, but we will limit our discussion to just three. First, malathion is widely used to control insects that eat the leaves of agricultural crops; it also is highly toxic to much-needed pollinators such as honeybees and can affect fish and birds as well. Second, the herbicide atrazine is a very effective way to kill weeds because corn is resistant to its toxic effects. Unfortunately, though, despite what might be our expectations of an herbicide, atrazine can adversely affect animals such as frogs by disrupting their reproduction. Third, the insecticide DDT was widely applied to farms and elsewhere until it was banned in the United States in 1972 (although it is still used to some extent around the world). Its low cost and effectiveness made it a popular choice. Due to a combination of its chemical properties and unexpected behavior in natural systems, however, it was ultimately linked to the deaths of large predatory birds such as bald eagles. More on DDT and the limitations of selective toxicity—including how an insecticide can affect eagles—may be found in Chapter 15.
Water and soil can be polluted
Pesticides that do not remain on their targets move into soil and water. Once there, they can affect soil communities, including those made up of microorganisms, and flow to ground and surface waters. The herbicide atrazine we saw in the previous paragraph, for instance, has been found in some drinking water reservoirs, raising concerns about the human health effects it can cause.
Insects can develop resistance to pesticides
A pesticide kills organisms susceptible to its toxic effects. However, it also provides an advantage to those few individuals unaffected by it. As we encountered in our discussions of antibiotic-resistant bacteria (e.g., Box 5.6), and evolution (Chapter 6), a population can quickly change from one sensitive to a pesticide like DDT to one that can survive in the presence of that same toxin. Since they reproduce much more quickly than humans (but slower than bacteria), pesticide-resistant insects can emerge within a few years of the first use of the compound.
Alternatives to synthetic pesticide use. Acceptable levels of pest control often can be achieved through means other than the use of chemical pesticides. Mostly because of issues related to cost and convenience, though, a large majority of farmers do not rely exclusively on these strategies.
Biological control
In some cases, farmers can take advantage of natural predator-prey relationships to reduce the number of insects eating their crops. They can either create and maintain environmental conditions that favor predators of pests or deliberately import such organisms onto their farms. For example, an extremely small wasp known as Trichogramma is often used to kill unwanted caterpillars through a form of parasitism. Lady beetles and predatory wasps are also effective biological control agents (Figure 9.17).
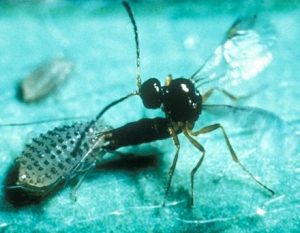
Crop rotation
This practice was described in the context of fertilizer use (review Figure 9.16), and it is also helpful in pest control. If done carefully, annual shifting of crops among different fields will limit the amount of useable food available to the insect young left behind from a previous growing season.
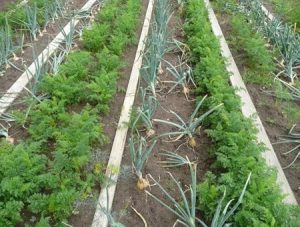
Intercropping
Instead of a single plant in one large field, several crops are cultivated in alternating rows within the same field (Figure 9.18, left). As a result, the advantages monoculture provides to insects are reduced.
Removal by hand and physical barriers for weed control
Weeds can be physically pulled out of the soil by farm workers, a practice that persisted for centuries prior to the wide-spread availability of herbicides. This very labor-intensive approach is not practical on large farms, but it can be seen on smaller scales. Alternatively, covering uncultivated soil between plants with tarps and similar objects is a very effective means to prevent weed growth, but it too is most relevant for small farms.
Integrated pest management (IPM)
Rather than relying on a single strategy to control pests, IPM combines many practices to reduce, but not eliminate, the use of chemical pesticides. The relative importance of the different tools—biological control, intercropping, crop rotation, pesticides (both synthetic and biopesticides)—varies from farm to farm and depends on local conditions. Farmers using IPM generally look at whole ecosystems rather than just a single species to manage pest problems.
Antibiotics limit the growth of disease-causing bacteria. The use of antibiotics is the final source of agricultural pollution on our list. Often, particularly on large, commercial farms, animals are fed antibiotics. At first, the strategy might appear to have some merit: money is lost if disease renders livestock (or their products) unfit for sale. In practice, though, widespread use of antibiotics comes with some serious risks. Specifically, and this story should sound familiar by now, bacteria have the ability to rapidly adapt to changing environmental conditions including the addition of poisonous substances. In the case of agriculture, antibiotics moving with animal excrement to soil and water encourage the development of antibiotic-resistant bacteria in those natural systems. Since some affected populations are likely pathogens, people could be sickened by diseases that cannot be easily cured. Livestock animals we wish to protect are similarly at risk.
9.4.4. Desertification
Defined simply, this is the process whereby a desert is formed, through natural or anthropogenic forces, in a temperate or other biome.
Causes: less water is available
Given what we know about environmental conditions in desert biomes (Chapter 5), it should come as no surprise that desertification is triggered by reduced availability of water in an area. But just what is powerful enough to effect such a change in water supply? Although a number of forces and activities contribute, agriculture plays a substantial role in the process.
Reduced precipitation. Perhaps the most obvious reason for desertification is a lack of input of water via important pathways such as rain, snow, and the like. A great deal of geologic evidence supports the notion that climatic conditions, including rainfall patterns, have changed throughout Earth’s history. Similar natural forces surely are active today as well, although human activities are also accelerating climate change. As a result, some temperate areas are becoming drier (among other effects). We will encounter this and related topics again in Chapter 14.
Reduced water-holding capacity. Since the amount of organic matter present (SOM) in soil is directly proportional to water-holding capacity, anything that removes SOM will also lower water availability to soil organisms. So, repeated farming of land can speed up topsoil erosion and yield increasingly sandy soil with time. Even if the amount of precipitation falling to the ground does not change, less water will stay around after rainstorms end, depriving plants of a vital resource. Then what happens? Well, as less and less vegetation is present, the soil becomes more and more vulnerable to erosion—fewer roots means less stability, as we know—and even more topsoil is lost. Future generations of crops will be even less healthy, more soil will be lost, and it goes on and on until all that remains is a desert (Figure 9.19).
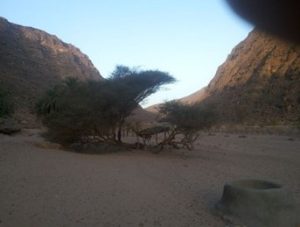
Desertification is generally considered to be a regional phenomenon because as a local farm becomes more of a desert, larger-scale precipitation patterns tend to change, causing surrounding non-farm areas to undergo similar transformations from relatively wet to relatively dry. Yet again we see the nature of positive feedback: the system responds to its own output with accelerated rates of change. In this case, as a farm starts to become a desert, the rate at which this shift occurs increases and the size of the area affected grows. If we expand our view further, we can see how erosion and desertification are connected to the growing human population, demand for food, and number of large, commercial farms. In short, this much larger system is likely to be characterized by positive feedback for the foreseeable future.
9.4.5. Redistribution of water
Agriculture accounts for about 40% of domestic freshwater usage in the U.S.[3][4]. Irrigation, especially in relatively dry climates such as southern California, can withdraw enormous quantities from surface and groundwater reservoirs and lead to shortages and conflicts among various interested parties. We will return to the complex issue of water usage and its consequences in Chapter 11.
9.4.6. Redistribution of carbon
This is the first of two global effects of agriculture on our list. Here, we briefly examine some of the ways farming increases the rate at which carbon (as CO2 gas) moves from the lithosphere to the atmosphere (see Chapter 14 for more on this topic).
Increased rates of decomposition
Since the environmental conditions inside undisturbed, dense soil clusters are not conducive to decomposition, organic carbon can persist in the lithosphere for centuries. Once a plow breaks soil into small and loose fragments, though, fungi and bacteria can quickly go to work and convert that stored carbon to CO2 gas.
Use of fossil fuels
Modern agriculture, most notably that carried out on large farms, uses an enormous amount of fuel to power mechanized equipment (tractors, bailers, and others). The organic carbon compounds in those petroleum products (typically gasoline and diesel fuel) are burned, and the resultant carbon dioxide moves into the atmosphere (Chapter 10). Fossil fuels are also used in pesticide synthesis and application, although they are not necessarily immediately converted to inorganic carbon during these processes.
9.4.7. Changes in Earth’s albedo
This is the second of our two global concerns. As we noted in Chapter 4 (section 4.3), the exposed, brown soil and small plants typically grown on farms reflect less energy than do the forests that were likely cleared to make room for agriculture. On a small scale, the effect of farming or gardening on albedo is trivial. However, large, continental-scale changes to land usage and surface properties could ultimately increase the amount of energy absorbed, as well as the average temperature of the whole Earth, in appreciable ways. See Chapter 14 for more.
9.5. GENETIC MODIFICATION OF ORGANISMS
We learned in Chapter 6 that genetic information is stored in DNA and that the specific codes carried in this molecule control the traits of all organisms, including crops and livestock. Agricultural scientists and farmers take advantage of the fact that DNA can be modified to increase our ability to produce food in many ways. Some of these practices are old, time honored, and trusted whereas others are new, relatively untested, and the cause of much debate.
9.5.1. Desirable traits are encouraged
Recognizing that individual organisms vary in their suitability for agriculture, farmers have been optimizing food production by manipulating genetic characteristics in their crops and livestock for centuries. What they do is related to, although not the same as, the process of natural selection we encountered in Chapter 6. Recall that individuals that are the most fit for survival are the ones that tend to reproduce, and the characteristics of subsequent generations will reflect the traits that maximize the chances for survival. Agricultural practices artificially manipulate which individuals are allowed to pass on their traits to the next generation. The ability to survive is not necessarily the most important trait to a farmer, rather, other advantages they bring to food production will be favored instead. Practices used to manipulate genetic characteristics and improve agricultural products can be divided into two groups: those that are conventional and widely accepted and those that are newer and controversial.
9.5.2. Approaches to genetic manipulation
Conventional genetic engineering
Selective breeding. A farmer could notice that a few members of a population grow faster, bigger, or otherwise better than the bulk of the others and then intentionally produce offspring from those most desirable individuals. Those offspring would likely have the characteristics of interest and would also be selectively bred until the whole population has been converted.
Cross breeding. Two individuals might each have some, but not all, traits that are desirable—say, one grows unusually large and the second produces uniquely sweet fruit. Offspring possessing all the advantageous characteristics could be produced from crossing these two plants. There are many examples of this practice, only two of which are noted here. First, the many varieties of different apples available for purchase at a supermarket have not always been present on Earth. Instead, they were produced by combining plants with different desired attributes. Other crops like corn and tomatoes have been similarly developed through this practice. Second, Holstein cows, the black and white ones generally used for dairy production in the U.S., are not altogether natural organisms. They are the result of centuries of cross breeding among different types of cattle to produce individuals with optimum traits. The Holstein has been specifically bred for maximum milk production, not necessarily for its meat (although those cows eventually end up slaughtered in any case).
New approaches to genetic engineering using biotechnology
Biotechnology is a general term that refers to various kinds of manipulations of organisms for the purposes of agricultural, industrial, commercial, or medical gains. In the context of agriculture, it can be used to affect specific DNA sequences controlling certain physical traits of crops and animals. Put into commonly used language, biotechnology can help produce genetically modified organisms (GMOs). Unlike cross breeding and other practices which require the relevant organisms to have enough in common that they could plausibly produce offspring without human intervention, these new methods can combine genes from unrelated organisms, those that would not be able to breed under natural circumstances. Genes from many organisms have been combined using these relatively new techniques. For example, plants have been crossed with each other, animals, and even bacteria. The use of biotechnology on farms is a very complex subject featuring both scientific rigor and controversy. Since exhaustive treatment of the topic would require much more space that we have available, we will focus attention on a few major points.
Potential benefits. The reasons GMOs were first developed and continue to be used are numerous. Generally, genetic manipulation that employs biotechnology is desirable because it allows for more targeted and faster modifications than do traditional cross breeding and related practices. A few specific examples of the kinds of advantages that can be realized through this practice are described here.
Crops can fight insect pests
A piece of bacterial DNA can be inserted into the genomes of corn (and others), giving plants the ability to produce insecticides on their own. One application of this idea resulted in a crop commonly referred to as “Bt corn”, so called to reflect how it was combined with the prokaryote Bacillus thuringiensis. We will return to this case in the section about risks of biotechnology, below, but for now note the benefit of this practice: the need for synthetic pesticides is greatly reduced on such farms.
Crops can survive herbicide exposure
Genes providing resistance to the toxic effects of glyphosate (the active ingredient in Roundup) have been transferred from bacteria to some agricultural crops. Now weed control is relatively simple: crops are the only plants left alive after fields are blanketed with the herbicide.
Nutrient content of crops can be enhanced
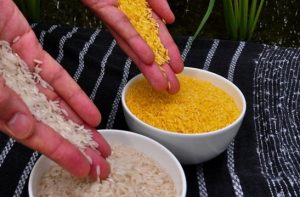
Genes from daffodils and a certain soil bacterium were inserted into the genome of rice to increase the amount of beta carotene in the crop. The result is a yellow product known as “Golden rice” (Figure 9.20). This modified rice has supplemented the amount of vitamin A available to people in areas that lack the critical nutrient.
Crops could withstand stresses of drought and temperature
Research into ways to use biotechnology to increase survival and success of plants under various hostile environmental conditions is ongoing. Since low water availability often limits crop yields, genes from desert plants could be combined with those of corn, rice, or other crops. Similarly, freezing can be a problem with tomatoes and other sensitive plants. Again, genes from plants that have adapted to survive the cold—like those from northern regions—could be inserted into the genomes of crops to produce more robust, resistant crops.
Risks, concerns, unanswered questions. Given the potential benefits described above, along with many others not mentioned, why are some people opposed to the use of GMOs? Several concerns, both scientific and otherwise, are repeatedly cited.
Risks to human health?
Put simply, people worry about the adverse effects that ingestion of GMOs can cause in consumers. Novel DNA sequences lead to the production of new, and potentially toxic, proteins by plants. In principle, negative consequences could range from mild allergic reaction to death. Various governmental agencies do regulate GMO use in human food to protect public health, but not everybody is satisfied. In the United States, genetically modified crops can be used in the production of both human food and animal feed, but it is illegal to apply biotechnology in the modification of animals used to make human food. Under certain circumstances, these products must be evaluated and shown to be safe, but many observers question the rigor, validity, and objectivity of the tests used (among their concerns: much of the research is conducted or funded by companies that profit from the sale of GMOs). Critics also note that food is not required by law to be labeled to reflect its GMO content, noting that consumers ought to be fully informed as they make nutritional choices. Elsewhere in the world, particularly in Europe, prohibitions against the use of GMOs are much stricter.
Risks to farms and natural environments?
Because of the number of published studies suggesting that GMOs present a small risk to human health, many scientists focus more on the potential threat that GMOs pose to Earth’s natural systems. Many concerns and unanswered questions remain.
Transfer of advantageous traits to weeds. Since genes can be passed among organisms through means other than reproduction, the ability to resist herbicides and other stressors might be transferred to unwanted plant species. The resulting organisms, sometimes referred to as “super weeds”, would be formidable competitors, potentially taking over farms as well as natural ecosystems. Changes in ecosystem structure would surely follow, including lowered biodiversity and damage to herbivores that rely on certain plant species for food (see Chapter 5). This phenomenon is poorly understood, and more research needs to be conducted to improve our knowledge about it.
Development of pesticide resistance. In the section on potential benefits of GMOs, above, we saw that Bt crops could produce their own insecticide. However, as with synthetic pesticides, insects have the ability to adapt to it; a population can quickly evolve to one dominated by individuals able to survive the toxin produced by the plant. Weeds can also become resistant. For example, extensive use of Roundup will select for individuals able to survive in its presence, possibly rendering the herbicide ineffective in the future. Agricultural scientists are aware of these problems and have been working on ways to maintain populations that are sensitive to the pesticides used against them, but success in this area is not guaranteed.
Effects on non-target organisms. In addition to its role in the development of pesticide resistance, the use of Bt crops raises another question: does the pesticide produced by plants harm organisms other than pests? As we know from earlier discussions, pesticides are not completely selective and often harm beneficial insects. Bt toxin is the same. Initially, concerns were raised that Monarch butterflies were being killed because corn pollen grains carried on the wind to nearby milkweed plants (a Monarch favorite) contained the toxin in them. Subsequent research demonstrated little evidence for the specific Br corn-milkweed-butterfly connection, but the larger issue about non-target effects remains unresolved.
The effects of sterile seeds
Traditionally, crops were sown and harvested, and seeds were collected from them at the end of the season to be used in the next year. These days, if they wish to use a genetically modified crop, farmers typically must purchase the seeds every year. Why is this? Because GMO crops are manufactured to be sterile, that is, they will not produce useable seeds when they grow (laws prohibiting the unauthorized acquisition and use of GMO seeds are also on the books). Farmers and their advocates cite this as exploitative and inappropriate because large corporations gain too much control over our food supply. The companies that develop the seeds counter that they have a right to dictate what happens to their products and to profit from their work. Furthermore, they say their seeds will perform more predictably and reliably if the randomness that comes from reproduction is not introduced. Like many others related to GMOs, this subject continues to be a source of conflict.
Other, values-based, arguments
For reasons we have seen in detail elsewhere, these are outside the scope of science. People often try to use them to sway their opponents, though—particularly when it comes to GMOs—so you should be cautious to evaluate if what you hear or read is based on measurable, objective data or subjective ideas about what ought to be true. Also remember that basing one’s actions on opinions, feelings, or convictions is not necessarily wrong, but it most certainly is not science.
What happens next? Genetically modified organisms are already being used extensively in agriculture. What the future holds depends on several factors, including our ability to meet demand for food, the work of advocates on both sides, public opinion, and our understanding of the risks and benefits of GMOs. Some people dismiss the idea of ever using genetically modified organisms on principle and will fight against them no matter what—some will even resort to sabotage, deceit, and intimidation. Others are ready to embrace GMOs under any circumstances, despite evidence that might warrant caution, using any means necessary to further their agenda. Scientists try to remain objective and are waiting for more data before making final assessments about the advisability of GMO use.
9.6. APPLYING OUR KNOWLEDGE: APPROACHES TO AGRICULTURE
Now that we have learned about agricultural processes and practices, we can briefly explore and compare some different ways food is produced.
9.6.1. Farming is different today than in the past
Since its beginnings many millennia ago, farming practices have changed a great deal. For example, both the size and scope of farms have grown enormously. People once kept small farms solely to feed their families (i.e., subsistence farms), but modern agriculture has become a large-scale industry employing millions of people to meet ever-growing global demand for food. The past century or so in particular has seen dramatic changes. According to the United States Department of Agriculture (USDA)[5], average farm size increased from about 200 acres in 1900 to about 440 acres in 2012, although American farms these days range in size from fewer than 10 to nearly 1,000,000 acres (globally, the biggest is about 6,000,000 acres). At the same time, fewer farms have become proportionally more important: today, only the largest 4% produce about two thirds of farmed food in the U.S. During the past few decades, though, there has also been an increase in the absolute number of small farms, perhaps indicating a growing interest in a return to local food production.
9.6.2. Some common types of contemporary farms
Farms vary in many ways, including size, type of food produced, strategies used to grow organisms, application of chemical additives, and use of genetically modified organisms. The earlier sections of this chapter present detailed information about the methods described below; consult them as necessary.
Conventional
This is a broad and diverse category of farms that currently produce most of the food in the United States.
Size. These facilities range from small, keeping just enough animals to feed the family living there (known as “rural-residence farms” by the USDA) to intermediate in size, up to a few hundred animals, to commercial farms, those that hold 500 or more cattle (or 1000s of chickens) at any one time (note that the actual maximum numbers in this last category can be very much higher, a hundred thousand or more animals in some cases). Tractors and other machines powered by fossil fuels (e.g., gasoline) are widely used.
Types of food. Plants directly eaten by humans and animals are produced here. Some common crops include corn, soybeans, and wheat. Livestock for human consumption such as beef, swine, and poultry are widely raised on conventional farms as well.
How food is grown. Again, acknowledging that farms differ, we make some broadly applicable observations.
Crops
Land clearing, plowing, crop rotation, and monoculture tend to dominate.
Livestock
Animals tend to be raised in confined spaces (e.g., pens and cages) often referred to as feedlots. Other practices can be used, though, including allowing animals to graze freely or simply have more space to roam (see Figure 9.21).
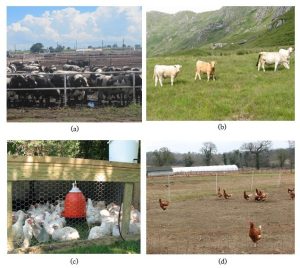
In any case, animals generally are fed large amounts of food and grown as quickly as possible. The waste produced can be used as fertilizer, but it also can move and pollute water. Finally, once they reach maturity (about two years for cattle, two months for chickens), the animals are slaughtered and their meat sold. Some, like dairy cows and chickens, are kept for their products, milk and eggs, respectively. They will likely be killed for their flesh, too.
Use of chemical substances. Nutrient deficiencies in fields are often mitigated through the application of synthetic fertilizers or manure, herbicides and insecticides are widely used to minimize losses, and hormones and antibiotics may be added to the diets of livestock.
Genetically modified organisms. Crops and livestock modified using biotechnology are often used.
Costs and benefits. We have seen the ways the practices used by conventional farmers provide both advantages and risks to human and environmental health in the earlier sections of this chapter. A point worth stressing here, though, is that food produced by conventional agriculture is nearly always less expensive for consumers to buy than that produced by organic means (described immediately below). Those who consume it either have decided its risks are acceptable or simply cannot afford more expensive products.
Organic
A farm can be certified as “Organic” by the USDA if it is operated according to an extensive set of regulations that dictate the conditions under which food must be raised (keep in mind that the conventional farms described above are not bound by these rules). We will refer to some of those items here, but the full list can be accessed on the USDA website[6].
Size. Farms here tend to be smaller—in some cases, by a great deal—than conventional farms. There is no size limit to be certified organic, but the type of work done on such farms is often most realistic at a smaller scale. Tractors and similar equipment are used, but more of the work is done by hand than is practical on large, conventional farms.
Types of food. The same kinds of food produced on commercial farms can be grown on organic farms. In addition, many of these small farms raise specialized plants, herbs, spices, and the like, for niche markets.
How food is grown.
Crops
Soil conservation methods tend to be more common on smaller organic farms. No-till agriculture and cover crops, for example, are often seen. Land clearing and plowing are still common, though.
Livestock
Animals are not fed food treated with synthetic chemicals and are also generally given far more space per individual than is seen on conventional farms. Organic farmers often strive to maintain animals in conditions that mimic natural ecosystems—so small cages and large feedlots are rare.
Use of chemical substances. The USDA standards for organic farming contain a list of allowed and prohibited substances, and farmers wishing to receive and maintain their certification must carefully adhere to the rules. Importantly, synthetic fertilizers and pesticides are prohibited, as are antibiotics and hormones.
Fertilizers
The application of animal manure and compost (See Box 9.1 for more on compost) to fields are common strategies used to replenish nutrients lost from soil.
Box 9.1. A few words about composting
Many farmers and gardeners use compost as a source of nutrients for the plants they grow. Just what is it? Put simply, compost is a nutrient-rich material derived from organic products such as scraps of uneaten vegetables, lawn clippings, leaves, and even certain paper and clothing waste. If these materials are exposed to the proper conditions, the natural process of decomposition will transform them into useable fertilizer. So, for example, the waste products can be put into a container of some kind (structures specifically designed for this purpose can be purchased through commercial vendors) along with some soil and specially adapted earthworms and / or other insects. Once it is closed up, bacteria and fungi quickly go to work and convert the strawberry tops, potato skins, and all the rest into unrecognizable organic residues that combine to create a substance very much like the soil organic matter we encountered earlier. In some cases, the compost is ready to spread on a field within a matter of months. Why is it favored by certain people? First of all, composting can be used to recycle useable organic compounds that would otherwise be thrown away and lost to a landfill. Secondly, it is a way to replenish soil nutrients without using expensive and potentially risky synthetic fertilizers. We will encounter composting again in our discussion of waste management (Chapter 13).
Pesticides
Since synthetic chemicals are prohibited on these farms, one or several alternative methods are used to control unwanted organisms.
Weeds. Three practices are often used: removal of weeds by hand, physical barriers such as tarps between rows of crops, and physical disruption of roots by tilling or plowing.
Insects. Biological control, crop rotation, and intercropping are generally employed by organic farmers to keep these pests from destroying their crops.
Genetically modified organisms. Organisms manipulated using biotechnology (i.e., GMOs) are prohibited on organic farms. The use of plants and animals that have been modified and selected through cross breeding and hybridization are allowed, though.
Costs and benefits. One of the goals of organic farming is to minimize the environmental impact of food production. The prohibition against synthetic chemicals and antibiotics certainly reduces pollution of water and the development of antibiotic-resistant bacteria, respectively. It also tends to slow the rate of erosion through its use of soil conservation methods. Both of these outcomes clearly are good for human and environmental health alike. Whether consumption of organic produce is appreciably better for humans than is eating alternatives is a matter of some uncertainty and debate, however. Some have argued that organic produce has a higher concentration of nutrients in it than does conventional, although scientific data have not convincingly demonstrated any such link. Does it taste better? Again, no definitive, objective evidence can be cited to support any claims that it does. The consumption of fewer pesticides, antibiotics, and hormones likely has a positive health impact, but the magnitude of this effect still needs to be rigorously quantified. Finally, given USDA regulations and the increased amount of labor required to grow it, organic food is more expensive to consumers than are produce and meats grown through conventional means. Many people choose to spend more, though, because they feel its benefits are worth the extra costs.
Blends, intermediates, combinations
Conventional and organic are not the only possible types of farms, but they are often held up as two opposite ends of a spectrum. Whether or not that characterization is completely fair or accurate does not change the fact that farmers can adopt a mix of strategies in response to their philosophies, goals, access to resources, knowledge, and experience. So, for example, the owner of a small farm might elect to use soil conservation methods, only use compost for fertilizer, raise free-range chickens, but also apply small amounts of synthetic pesticides to control insects. Although not certified by the USDA, it would closely resemble an organic farm. On the other hand, large commercial farmers can adopt various practices usually associated with organic agriculture such as no-till cultivation, cover crops, and the use of large spaces for livestock to roam and feed.
9.6.3. Other, less common approaches
Mounting demand for both space and food has driven the development of many creative strategies to grow food. The historic notion of subsistence farming (mentioned in 9.6.1) appeals to certain modern people, even those who live in densely populated areas. Among other approaches used are community gardens (spaces shared by multiple families), greenhouse, rooftop (exactly what the name suggests!) and vertical (various structures are used to minimize the need for extensive horizontal space, see Figure 9.22) farming. Such strategies can certainly yield small amounts of edible crops but are difficult to scale up.
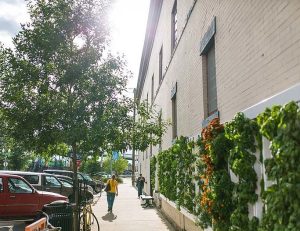
9.7. WHAT IS THE FUTURE OF AGRICULTURE?
As we know, Earth is closed with respect to materials like biologically available forms of nutrients, fresh water, fertile soil, and farmable land. How, then, will we be able to keep up with rising demand for food with no increases in the resources necessary to do so? Clearly, several issues must be resolved if we are to be successful.
9.7.1. More efficiency
Production of more food in the space currently available will be necessary. In other words, crop yields will need to increase somehow. Genetic modifications, either using traditional practices or biotechnology, may be part of the solution here. Issues regarding human and environmental health need to be fully researched and addressed, though, before GMOs would likely be widely accepted or even practical (as noted earlier).
9.7.2. Change in consumption habits
As noted in Chapter 8, both the total number of individual humans on Earth and the rate of resource use per person are likely to rise into the foreseeable future. In principle, a reduction in the number of excess calories consumed would reduce some of the stresses of agriculture, but in practice such a goal will likely be elusive.
9.7.3. Soil conservation
Topsoil loss is an enormous problem, one that, arguably, receives far less attention and concern than it merits. As we saw, current rates of erosion outpace rates of soil formation, so changes in strategy will be needed to ensure our ability to farm into the future. If things continue on their current trajectory, Earth’s agricultural fields could be effectively barren within a century. Ready to feed that 11 or so billion people with no fertile soil?
9.7.4. Food production without soil?
It is possible to grow plants in water solutions, that is, hydroponically. Currently, the amount of food produced in this way is relatively trivial, but research in the area may increase its feasibility. Unless soil loss is slowed considerably, this and other soil-free growing techniques may be required in the future.
THE CHAPTER ESSENCE IN BRIEF [7]
Agroecosystems are used to produce human food. Although specific approaches to farming vary, all harness natural ecological processes and rely on knowledge of soil science. Agriculture tends to cause adverse environmental consequences and is associated with controversial uses of pesticides, fertilizers, and biotechnology.
Think about it some more…[8]
What variables influence the suitability of a soil to support crop production?
If nitrogen is so abundant in the atmosphere, how can it ever be a limiting factor for crop growth? Why do farmers often add nitrogen fertilizer to fields?
How could agriculture contribute to mass wasting (Chapter 7)?
Remembering that Earth is closed with respect to materials, what do you think is the best way to meet the ever-increasing demand for food by an ever-growing human population? Are any of the farming practices described in Chapter 9 out of the question for you? If yes, what are the alternatives?
- US Department of Agriculture (USDA). Soil classification. https://www.nrcs.usda.gov/resources/guides-and-instructions/soil-classification ↵
- Pimentel, D, Burgess, M. 2013. Soil erosion threatens food production. Agriculture. 3:443‒463 ↵
- US Geological Survey. 2019. Total Water Use. https://www.usgs.gov/mission-areas/water-resources/science/total-water-use?qt-science_center_objects=0#qt-science_center_objects ↵
- The term 'sector' is used to indicate a class of water users. For example, the agricultural sector includes farms and is the largest user of water. The power generation sector can use a lot of water as well, although the amount varies from place to place. The industrial and domestic sector (homes) tend to use much less water than the others. Consult Chapter 11 for more about sectors and water usage. ↵
- Farm Size and the Organization of U.S. Crop Farming. 2013. USDA Economic Research Service. Economic Research Report Number 152 PLUS 2012 USDA farm census ↵
- https://www.ams.usda.gov/rules-regulations/organic ↵
- As you will find throughout this book, here is very succinct summary of the major themes and conclusions of Chapter 9 distilled down to a few sentences and fit to be printed on a t-shirt or posted to social media. ↵
- These questions should not be viewed as an exhaustive review of this chapter; rather, they are intended to provide a starting point for you to contemplate and synthesize some important ideas you have learned so far. ↵
In ecology, organisms that are most like pioneer species in their requirements. They tend to grow in relatively barren habitats. See Chapter 5 for details.
In ecology, a type of ecosystem. Depending upon who you ask, Earth has between 5 and 15 biomes, and each is characterized and classified according to dominant organisms present and environmental conditions. See Chapter 5 for details.
In geology, a term that refers to chemical and physical processes that break down and transform rocks and the remains of living materials. Weathering products can be transported and incorporated into new rocks and soils. Compare to erosion. See Chapter 3 for more.
A general word that refers to the breaking down of relatively large, complex materials into relatively small, simple materials. Certain organisms, known as decomposers, break down the remains and waste products of other organisms and use those products as nutrient sources. Decomposers are critical to the health of ecosystems because they aid in the recycling of materials. Fungi and some bacteria are important decomposers. See Chapters 4 and 5 for more.
In chemistry, refers to chemical compounds that lack at least one of the following: a C atom and at one C-H bond. Compare to organic. See Chapter 4 for details.
In chemistry, when a neutral atom gains or loses electrons, thereby gaining or losing negative charge, it becomes a charged ion. Loss of electrons produces a positive ion, gain of electrons produces a negative ion. See Chapter 4 for more.
In agriculture, the largest amount of a crop that can be sustainably grown in a field. See Chapter 9 for more.
Equal to the number of individuals of a species that can be sustainably supported by an ecosystem. See Chapter 1 for more.
An example of ecological succession that transforms a barren sand dune into a small forest. Among other changes that occur with time: the dune is stabilized by grasses specifically adapted to grow in unstable sand. See Chapter 5 for details.
In ecology, refers to the specific identity of organisms present in an ecosystem. This property varies widely among ecosystems. See Chapter 5 for details.
A broad term that refers to the number of different organisms in an ecosystem or, often, the whole Earth. There are several specific ways to measure and express biodiversity. See Chapters 5 and 6 for details.
In a system, current output accentuates or magnifies the effect of previous output. This type of feedback leads to change away from initial conditions. Compare to negative feedback. See Chapter 2 for more.
Specialized bacteria that can convert dinitrogen into ammonium. They are crucial in providing biologically available N to most of the biosphere. See Chapters 4, 5, and 9 for more.
In ecology, a relationship among organisms in which all participants benefit. See Chapter 5 for details.
The process of converting C from non-biologically available forms to forms that can be used by organisms. Typically, carbon dioxide molecules are converted to glucose. C fixation is carried out by organisms that possess the proper machinery to do so: plants, algae, and some bacteria. Fixation is a general term that indicates an increase in the biological availability of any atom. See Chapter 4 for more details.
A process whereby a relatively clear aquatic system is transformed to first a murky then swampy then, potentially, a dry terrestrial system. It is caused by the accumulation of excess nutrients (nitrogen and/or phosphorus). See Chapters 4 and 11 for details.
A process carried out by certain organisms (plants, algae, and some bacteria) in which the energy of the sun is used to drive the fixation of carbon. See Chapters 4 and 5 for more details.
Both too little and too much of an essential nutrient can harm natural systems. See Chapters 4 and 15 for details.
A fundamental concept in the science of toxicology. Different species, and even different individuals from the same species, will respond differently to the same poisonous substance. See Chapter 15 for more details.
In ecology, a group of interacting populations. See Chapter 5 for details.
A population of bacteria that is no longer susceptible to the toxic effects of a particular antibiotic. Note it was once dominated by pesticide-sensitive individuals, but rapid evolution led to its ability to survive in the presence of the poison. See Chapters 5 and 6 for details.
Holds that populations change with time in response to a number of factors, including mutations, natural selection, and random chance. Biological evolution is the main scientific explanation for the development of life on Earth. See Chapter 6 for many more details.
In ecology, an association between organisms in which one, the predator, lives at the expense of the other, the prey. Predators consume prey. See Chapter 5 for details.
A gas made up of one carbon atom and two oxygen atoms, it is one of the many forms carbon can take. This gas is the product of aerobic respiration as well as combustion of fossil fuels, wood, and other materials containing organic carbon. It is not directly usable as a carbon source but can be fixed into useable forms of carbon, for example glucose, by autotrophic organisms. See Chapters 4 and 14 for more.
The amount of solar radiation reflected off any planetary body. See Chapters 4 and 14 for details.
Refers to all the information that can be used to copy cells. See Chapter 6 for more.
A biochemical molecule containing the code that makes every organism unique. See Chapter 6 for details.
In evolutionary theory, individuals that are best adapted to survive in their environments will reproduce better than those are less well adapted. See Chapter 6 for details.
A type of pesticide that specifically targets unwanted plants (i.e., weeds). See Chapters 9 and 15 for details.
A structure designed to contain waste. See chapter 13 for details.